Physiologic Basis of Fetal Monitoring
Clinical fetal heart rate (FHR) monitoring is an ongoing observation of human fetal physiology. The question being asked by the clinician is: What is the adequacy of fetal oxygenation? Because the FHR pattern appears to assume certain characteristics under the influence of various hypoxic and nonhypoxic influences, it becomes important for the clinician to have a basic understanding of the physiology of fetal respiratory exchange and the physiologic control of the FHR. In this chapter, we outline the principal factors involved in fetal oxygenation and carbon dioxide transfer as well as the basis for our current understanding of FHR responses to changes in fetal respiratory status.
THE ANATOMY OF MATERNAL-FETAL EXCHANGE
The placenta is an organ that functions as the fetus’ extracorporeal life-support system. The placenta serves as the fetal lung (respiration), kidney (excretion), gastrointestinal tract (nutrition), and skin (heat exchange) and as a barrier against certain substances dangerous to the fetus. In addition, it is an endocrine organ that produces steroid (estrogen, progesterone) and protein (human chorionic gonadotropin, human placental lactogen) hormones. Very early in gestation, the blastocyst implants in the decidualized endometrium and the trophoblast cells invade the maternal circulation, creating a lake of maternal blood that bathes the trophoblast and developing embryo. As the gestation grows, a number of spiral arteries that supply blood to the endometrium are penetrated and provide the basic architecture as the placenta develops, with villi forming cotyledons arranged around these spiral arteries. The fetal chorionic villi develop many convolutions and float in the maternal blood supplied from the previously invaded spiral arteries. This maternal blood occupies an area referred to as the intervillous space, and it is between this space and the fetal capillary (contained within the chorionic villus) that maternal-fetal and fetal-maternal exchange occurs. The human placenta is thus referred to as a hemochorial type because the mother’s blood comes into direct contact with the fetal chorionic villus (1). Oxygen, carbon dioxide, nutrients, waste products, water, and heat are exchanged at this level and must cross two layers of fetal trophoblast, the fetal connective tissue within the villus, and the fetal capillary wall (Fig. 2.1).
The uterine blood flow is supplied principally from the uterine arteries, but anastomoses occur between these vessels, other branches of the hypogastric arteries, and ovarian arteries. Significantly, the spiral arteries must traverse the full thickness of the myometrium to reach the intervillous space. Anything that affects maternal cardiac output will, of course, affect the flow through the spiral arteries. Additionally, as the
uterus contracts, the intramyometrial pressure may exceed the intraarterial pressure, causing occlusion of these vessels and resulting in cessation of blood flow to the intervillous space (Fig. 2.2) (2).
uterus contracts, the intramyometrial pressure may exceed the intraarterial pressure, causing occlusion of these vessels and resulting in cessation of blood flow to the intervillous space (Fig. 2.2) (2).
Approximately 85% of the total uterine blood flow goes to supply the placental (intervillous) circulation and 15% supplies the extraplacental uterine musculature (3). The intervillous space blood supplies the vascular support to the placenta itself, and if the blood flow to any area is sufficiently compromised, an infarction of the placenta may occur (4,5).
For clinical purposes, the intervillous space circulation is probably maximal when the mother is at rest, in the lateral position. Although estrogen administration to the pregnant ewe will increase uterine blood flow, it is not certain that this results in increased intervillous blood flow (5,6). Therefore, although we know of nothing that will increase effective uterine blood flow above that found in the resting lateral position, there are many things that will decrease uterine blood flow.
FACTORS THAT DECREASE UTERINE BLOOD FLOW
Position
Changes in the mother’s position may decrease blood flow to the uterus by at least two mechanisms. The supine position is characterized by an exaggeration of the lumbar lordotic curve, resulting in uterine compression of the vena cava, the aortoiliac vessels, or both. With vena caval occlusion, the return of blood to the heart is decreased, and this may result in a fall in maternal cardiac output, maternal hypotension, and decreased uterine blood flow (7,8). Compression of the aorta against the spine or iliac vessels as they cross the pelvic brim may result in decreases in uterine blood flow without maternal hypotension (Fig. 2.3). Abitbol et al. (9) showed that patients in the supine position had an increased incidence of late decelerations during labor and this correlated with a fall in femoral arterial pressure, a decrease in the amplitude of the big toe capillary pulse, and a drop in the fetal scalp blood pH, all of which were reversible when patients were returned to the lateral position. Simpson and James (10), using measurements of fetal oxygen saturation via fetal pulse oximetry, in a randomized trial, showed a 29% improvement in oxygen saturation with either lateral position compared to supine.
Exercise
Maternal exercise may serve to divert blood away from the uterus to supply somatic muscle groups, resulting in decreased uterine blood flow. Clapp (11) has shown that baseline FHR increases consistently following exercise in pregnant women. The tachycardia following exercise is not believed to be due to hyperthermia or increased fetal activity but rather is a sympathetic response to a period of reduced fetal oxygenation. Artal et al. (12) have described three cases of fetal bradycardia occurring during postexercise fetal tachycardia, suggesting that in those cases there was more profound hypoxia. These studies in humans tend to support the caution originally suggested in Emmanouilides et al.’s research into exercising pregnant sheep (13). Although there is evidence that exercise is not detrimental to the fetus where there is normal uteroplacental function (14), it is possible that some fetuses could be adversely affected by excessive maternal exercise.
Uterine Contractions
The spiral arteries that traverse the myometrium are subject to collapse as the uterus contracts and the intramyometrial pressure exceeds the spiral arterial pressure. During normal pregnancy, the uterus has certain inherent contractility (Braxton-Hicks contractions), and as labor begins, the contractions increase in frequency and intensity. If the uteroplacental oxygen exchange is normal, the Braxton-Hicks contractions and labor contractions do not appear to significantly compromise the total intervillous space blood flow. However, a normal fetoplacental unit may have its uteroplacental reserve exceeded if uterine activity is excessive, as with spontaneous or oxytocin-induced uterine hypertonus and/or tetanic contractions (15,16). Patients with abruptio placentae may have hypertonus and tachysystole, resulting in decreased intervillous space perfusion producing fetal hypoxia. Orgasmic coitus has been reported to be associated with increased uterine activity and prolonged FHR deceleration in three patients studied with simultaneous FHR and uterine contraction monitoring (17).
Surface Area
Anything that will decrease the effective surface area of the placenta will clearly increase the potential for fetal hypoxia. Abruptio placentae is a classic example of reduced placental surface area available for exchange. Patients with multiple placental infarcts, as may be seen in hypertensive disorders and prolonged pregnancy, are also subject to having the fetus suffer from uteroplacental insufficiency (UPI). There is recent evidence that hypercoagulable states in the fetus may result in thromboses of the fetal vessels in the placenta that may lead to growth restriction and decreased available oxygen to the fetus (18).
Anesthesia
The administration of conduction anesthetics carries the potential for reduced intervillous space blood flow secondary to maternal hypotension, resulting from the sympathetic blockade that may occur to a greater or lesser degree in all such patients. The pharmacologic correction of such hypotension with a-adrenergic agents may not restore uterine blood flow because the α-adrenergic agents will increase uterine circulatory resistance along with the other somatic components of total peripheral resistance that are responsible for the increase in blood pressure. For this reason, it is recommended that an agent such as ephedrine (a mixed α- and β-adrenergic stimulator) be used to restore maternal blood pressure after hypotension induced by the sympathectomy of conduction anesthesia (19). Usually, however, the uterine blood flow is easily restored in such situations by correcting positional factors and expanding maternal blood volume with increased intravenous fluids, thus obviating the need for any pressor agents.
Using isotope techniques, reports of increased uterine blood flow following epidural anesthesia have given rise to some questions regarding the classic understanding of sympathectomy caused by conduction anesthesia, resulting in diversion of blood from the uterus (20). Data using Doppler flow techniques have shown that diastolic flow in the arcuate arteries may actually increase in prehydrated patients following epidural anesthesia (21). The proposed mechanism involves a decrease in sympathetic tone in the uterine circulation, resulting in decreased resistance. It is not clear, however, whether this finding actually represents increased placental flow or merely opening of the nonplacental portion of the uterine circulation. The reason one must question data suggesting that epidural anesthesia increases uteroplacental blood flow is related to the frequent finding of late decelerations following epidural activation. Further research in this area is needed to clarify the exact relation of conduction anesthesia to uteroplacental blood flow.
Hypertension
Maternal hypertensive syndromes may result in decreased intervillous space blood flow as a result of either acute vasospastic or chronic atheromatous changes in the uterine arterial blood supply. If one lowers blood pressure in the hypertensive patient either intentionally with antihypertensive agents or unintentionally as a result of administering a conduction anesthetic, one runs the risk of diverting blood away from the intervillous space if the caliber of the uterine arterial circulation remains diminished as other vascular beds are dilated.
Diffusion Distance
The thickness of the placental membrane between the intervillous space and the fetal capillary may also decrease the transfer of oxygen. An example of a clinical entity demonstrating this phenomenon can probably be found in fetal erythroblastosis with placental edema. Perhaps this is also a factor in certain conditions of fetal dysmaturity where there is an increase in fibrin deposition between the intervillous space and the fetal capillary. Villous hemorrhage and edema in diabetics may also play a role in increasing the thickness of the placental membrane.
THE FETAL CIRCULATION
The anatomy and physiology of the fetal circulation are very complex. For the purposes of this chapter, we focus on the umbilical circulation and factors that affect placental exchange (Fig. 2.4).
The umbilical vessels are contained within the umbilical cord and are protected with an abundance of a substance
called Wharton’s jelly. Normally, there are two umbilical arteries that arise from the terminal ends of the fetal hypogastric arteries and a single umbilical vein that returns blood to the fetus from the placenta, channeling it partly through the liver and partly to the inferior vena cava via the ductus venosus. This well-oxygenated blood enters the right atrium and follows a course directing it mainly to the cephalic circulation, while blood returning from the upper body via the superior vena cava is channeled principally through the ductus arteriosus to the lower body and the placenta. Approximately 30% of the fetal cardiac output goes to the placenta, which comprises a low-resistance vascular bed. The oxygen content of the umbilical venous blood closely approximates that of the uterine venous drainage, which suggests that the pattern of umbilical and uterine flows functionally follows a concurrent relationship, although the flow relationships are clearly much more complex and authorities in the field are not in total agreement concerning this fact (22).
called Wharton’s jelly. Normally, there are two umbilical arteries that arise from the terminal ends of the fetal hypogastric arteries and a single umbilical vein that returns blood to the fetus from the placenta, channeling it partly through the liver and partly to the inferior vena cava via the ductus venosus. This well-oxygenated blood enters the right atrium and follows a course directing it mainly to the cephalic circulation, while blood returning from the upper body via the superior vena cava is channeled principally through the ductus arteriosus to the lower body and the placenta. Approximately 30% of the fetal cardiac output goes to the placenta, which comprises a low-resistance vascular bed. The oxygen content of the umbilical venous blood closely approximates that of the uterine venous drainage, which suggests that the pattern of umbilical and uterine flows functionally follows a concurrent relationship, although the flow relationships are clearly much more complex and authorities in the field are not in total agreement concerning this fact (22).
One must ask how the fetus can exist at a maximum pO2 below that of the uterine vein (about 35 mm Hg), whereas the adult would be unable to survive under similar circumstances. The fetus has a number of unique characteristics that allow it thrive with such a low pO2.
First, the fetal hemoglobin concentration is higher than that in the adult, allowing for a much greater oxygen carrying capacity. Second, the fetal cardiac output far exceeds that of the adult on a volume per unit body weight basis. Third, the fetal hemoglobin dissociation curve favors a higher saturation at a given pO2 (Fig. 2.5). So, although the fetal pO2 is lower, the fetus is able to compensate by having an increased oxygen content, due to the high hemoglobin concentration and the characteristic of the fetal hemoglobin dissociation curve. The more rapid circulation then increases the amount of oxygen that can be delivered to the fetal tissue per unit time (23).
Oxygen crosses the placenta by simple diffusion. If the relative flows on the two sides of the placenta are held constant, the differential pO2 between the intervillous space and the fetal capillary determines the rate of maternal-fetal oxygen transfer. Thus, administration of high concentrations of oxygen to the mother may increase her pO2 several hundred millimeters of mercury and the maternal-fetal oxygen gradient may be markedly increased by this maneuver (24). Moreover, if the fetal pO2 is increased by only a few millimeters of mercury, the fetal blood oxygen content may increase
significantly because, in either the physiologic or hypoxic range, the fetal hemoglobin saturation curve is quite steep (Fig. 2.5) (25).
significantly because, in either the physiologic or hypoxic range, the fetal hemoglobin saturation curve is quite steep (Fig. 2.5) (25).
Clinically, however, even though maternal hyperoxia maybe of some help, most causes of fetal hypoxia are related to restriction of umbilical or intervillous space blood flow. Under conditions of diminished flow on either side of the placenta, changing the pO2 gradient will not be of as much help as restoring the blood flow. It is still reasonable to administer oxygen to the mother (26) in such situations, but one must remember that restoration of flow is usually more important.
Fetal Circulatory Response to Hypoxia
Fetal circulatory responses to hypoxia involve redistribution of blood flow.
These include
A redistribution of cardiac output resulting in preservation of blood flow to certain vital organs, including brain, myocardium, and adrenal glands, at the expense of flow to certain less vital organs including the parathyroid glands, lungs, liver, kidneys, intestines, bone marrow, and somatic muscles
A loss of cerebral vascular autoregulation resulting in a pressure-passive cerebral circulation
An eventual decrease in cardiac output resulting in hypotension and ultimately a decrease in cerebral blood flow (27,28 and 29). Fetal cerebral vascular resistance can decrease by at least 50% and maintain cerebral blood flow allowing for only a minimal decrease in oxygen delivery. With persistent hypoxemia leading to arterial hypotension, cerebral vascular resistance cannot maintain flow by vasodilation, leading to a marked reduction in cerebral blood flow. This can then lead to neuronal necrosis in the fetal brain.
There are also noncirculatory responses to hypoxia that are important in preserving neuronal integrity under hypoxic conditions. These include
A slower depletion of high-energy compounds during hypoxia-ischemia in the fetus compared with the term infant or adult
The use of alternate energy substrate, the neonatal brain having the capacity to use lactate and ketone bodies for energy production
The relative resistance of the fetal and neonatal myocardium to hypoxia-ischemia
The potential protective role of fetal hemoglobin
Because of these circulatory and noncirculatory responses to hypoxia, the fetus has considerable protection from neuronal damage, and even with severe hypoxic insults, most fetuses that survive have little or no central nervous system (CNS) damage.
CONTROL OF THE FETAL HEART RATE
The FHR, under physiologic conditions, represents the final product of intrinsic and extrinsic rate-determining or rate-modifying factors. Technically, the FHR represents the reciprocal of the interval between two successive beats. Most data on FHR use an electrical marker (specifically the peak of the fetal electrocardiogram [ECG] R wave) to signify the time of the beat. Unless otherwise stated, we will refer to the rate calculated from intervals between fetal ECG R waves. FHR changes constitute the basis for electronic fetal monitoring, and for this reason, one must look carefully at the factors that determine or modify the rate.
Schifferli and Caldeyro-Barcia (30) discovered that the baseline heart rate decreases with gestational age. At 15 weeks’ gestation, the average baseline rate is approximately 160 beats per minute (BPM) (Fig. 2.6). Although premature fetuses may have a slightly increased rate over that found at term, within the limits of fetal viability (from 28 weeks to term), the average baseline FHR difference is only approximately 10 BPM. One must, therefore, be careful not to attribute a baseline tachycardia to prematurity when it may well be a sign of fetal compromise. Any baseline FHR above 160 BPM must be explained on some basis other than fetal prematurity.
Schifferli and Caldeyro-Barcia (30) further noted that if one administers atropine to a fetus, the resulting increase in heart rate is of greater magnitude as one approaches term, and that the postatropine heart rate is usually in the range of 160 BPM. Because atropine is a parasympathetic blocking agent, it would appear that the gradual decrease in FHR that
occurs with increasing gestational age can be explained as an increase in parasympathetic tone (Fig. 2.6).
occurs with increasing gestational age can be explained as an increase in parasympathetic tone (Fig. 2.6).
Renou et al. (31) have shown that upon administering atropine directly to a human fetus during labor, three phenomena are observed. First, there is a modest increase in the baseline FHR, presumably due to blocking of the tonic parasympathetic effect described by Schifferli and Caldeyro-Barcia (30). Second, Renou et al. noted a loss of the variability of the FHR, suggesting that a continuous balance between the parasympathetic slowing effect and the sympathetic accelerating effect may have been disturbed. Third, they noted that a majority of patients demonstrated the appearance of FHR accelerations during uterine contraction. This suggests that, before release of parasympathetic tone by atropine, the accelerating forces were present but suppressed. Renou et al. then added a β-adrenergic blocking agent (propranolol) to these atropinized fetuses and noted abolition of the accelerations and a decrease in the baseline FHR (Fig. 2.7). It
would thus appear that the accelerating forces unmasked by atropine blockade were of β-sympathomimetic origin. With this information, it is then possible to think of the baseline FHR as a product of the modulated influences of the parasympathetic and sympathetic nervous systems. Furthermore, the baseline FHR variability probably represents an instantaneous product of these two forces that are constantly working in a push-pull relationship; the presence of good FHR variability probably requires the integrity of these two modulating forces.
would thus appear that the accelerating forces unmasked by atropine blockade were of β-sympathomimetic origin. With this information, it is then possible to think of the baseline FHR as a product of the modulated influences of the parasympathetic and sympathetic nervous systems. Furthermore, the baseline FHR variability probably represents an instantaneous product of these two forces that are constantly working in a push-pull relationship; the presence of good FHR variability probably requires the integrity of these two modulating forces.
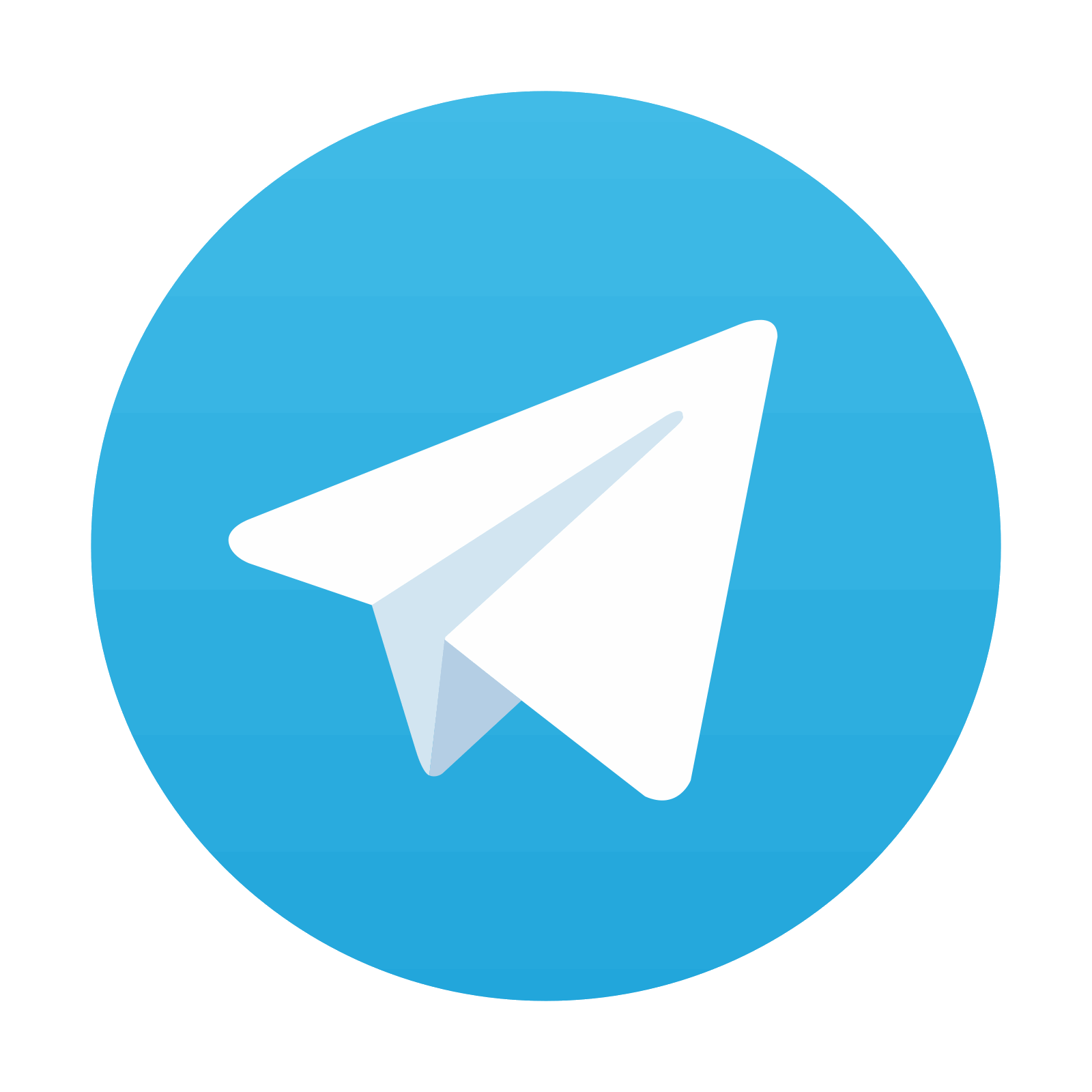
Stay updated, free articles. Join our Telegram channel
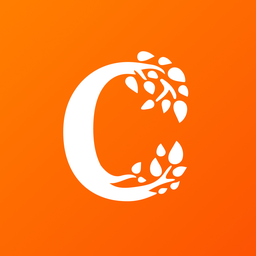
Full access? Get Clinical Tree
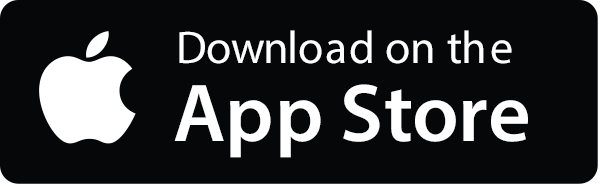
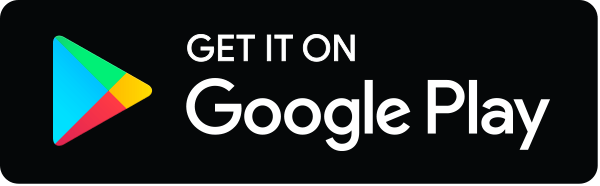