Pediatric Techniques and Vascular Anomalies
Orhan Konez
Katharine L. Hopkins
Patricia E. Burrows
Background and Importance
Pediatric vascular anomalies (commonly referred to as birthmarks or vascular birthmarks) are a heterogeneous group of lesions for which numerous classification systems have been proposed. The most commonly used classification system, based on endothelial characteristics of different lesions, is derived from work of Mulliken and Glowacki (1). It divides pediatric vascular anomalies into two categories (Table 23-1). Vascular tumors—including hemangiomas and hemangioendotheliomas—are proliferative endothelial cell neoplasms. Most hemangiomas tumors develop during infancy, proliferate rapidly, stabilize for a period of time, and then slowly regress (2). In contrast, vascular malformations are developmental anomalies of vasculogenesis and angiogenesis, composed of dysplastic vessels. They generally are present at birth, grow in proportion to the body during childhood, and do not involute. Vascular malformations are classified further according to flow characteristics—high or low—and predominant channel involvement—arteriovenous (AVM), capillary (CM), venous (VM), lymphatic (LM), or combined (2,3).
Morbidity associated with pediatric vascular anomalies is variable and is influenced by lesion type, size, location, and behavior. Mass effect may cause compression of vital structures, airway compromise, or visual impairment.
Other local complications such as ulceration, infection, hemorrhage, and ischemia may occur (4,5). Systemic effects, which may be life threatening, include high-output congestive heart failure, anemia, consumptive coagulopathy, and altered drug pharmacokinetics (2,4,6). In some children, diffuse vascular malformations are associated with inhibition or acceleration of bone growth, soft tissue hypertrophy, ectopic adipose tissue, and limb gigantism (7,8). Vascular lesions may also cause considerable disfigurement—either temporary or permanent—with secondary effects on self-image and psychosocial development. Many of the pediatric vascular anomalies occur as part of syndromes. Familial forms are less common than sporadic ones. Characteristics of individual lesions as well as frequently associated syndromes will be discussed in detail in this chapter.
Other local complications such as ulceration, infection, hemorrhage, and ischemia may occur (4,5). Systemic effects, which may be life threatening, include high-output congestive heart failure, anemia, consumptive coagulopathy, and altered drug pharmacokinetics (2,4,6). In some children, diffuse vascular malformations are associated with inhibition or acceleration of bone growth, soft tissue hypertrophy, ectopic adipose tissue, and limb gigantism (7,8). Vascular lesions may also cause considerable disfigurement—either temporary or permanent—with secondary effects on self-image and psychosocial development. Many of the pediatric vascular anomalies occur as part of syndromes. Familial forms are less common than sporadic ones. Characteristics of individual lesions as well as frequently associated syndromes will be discussed in detail in this chapter.
Table 23-1 Classification of Vascular Anomalies | |||||||||||||||||||||
---|---|---|---|---|---|---|---|---|---|---|---|---|---|---|---|---|---|---|---|---|---|
|
In total, pediatric vascular anomalies are quite common. Hemangioma is diagnosed in 10% to 12% of infants and children and is the most frequently encountered pediatric soft tissue neoplasm (3,4,8). Venous malformations, many of which are silent, are estimated to occur in up to 50% of the general population (9). Since some lesions result in greater morbidity and require more aggressive therapy than others, accurate diagnosis has important implications with respect to treatment and prognosis (2,4).
Imaging Strategies
Overview
Many pediatric vascular anomalies can be correctly diagnosed based on clinical presentation and physical examination alone; others require diagnostic imaging. The characteristic imaging features are summarized in Table 23-2. Sonography is often the first imaging study performed. Grayscale sonography, spectral analysis, and color Doppler ultrasound all have value in characterizing vascular lesions (5,10). The advantages of ultrasound are that it uses no ionizing radiation; requires no sedation or contrast administration; and is noninvasive, relatively inexpensive, and readily available. Disadvantages of ultrasound include limited soft tissue differentiation, poor echo transmission through air and bone, and operator dependence. Radiologists and sonographers who are inexperienced with respect to the sonographic evaluation of vascular anomalies may have difficulty differentiating the lesions.
Magnetic resonance imaging (MRI) and computed tomography (CT) complement sonography and, particularly when lesions are large or complex, provide additional information about soft tissue content and extent (3,10). Because of their excellent spatial resolution and
three-dimensional (3D) display capabilities, MRI and CT are superior techniques for defining positional relationships with respect to deep organs and vital structures. Magnetic resonance angiography (MRA) and computed tomographic angiography (CTA) may also be used to delineate feeding arteries and draining veins prior to interventional or surgical treatment (11,12). In patients with vascular syndromes, information may be gained from these modalities regarding associated anomalies as well. In many cases, invasive arteriography is only necessary for purposes of embolization (3).
three-dimensional (3D) display capabilities, MRI and CT are superior techniques for defining positional relationships with respect to deep organs and vital structures. Magnetic resonance angiography (MRA) and computed tomographic angiography (CTA) may also be used to delineate feeding arteries and draining veins prior to interventional or surgical treatment (11,12). In patients with vascular syndromes, information may be gained from these modalities regarding associated anomalies as well. In many cases, invasive arteriography is only necessary for purposes of embolization (3).
Table 23-2 Imaging Findings in Pediatric Vascular Anomalies | ||||||||||||||||||||||||||||||||||||||||||||||||||||||||||||||||||||||||||||||||||||||||||||||||||||||||||||||||||||||||||||||||||
---|---|---|---|---|---|---|---|---|---|---|---|---|---|---|---|---|---|---|---|---|---|---|---|---|---|---|---|---|---|---|---|---|---|---|---|---|---|---|---|---|---|---|---|---|---|---|---|---|---|---|---|---|---|---|---|---|---|---|---|---|---|---|---|---|---|---|---|---|---|---|---|---|---|---|---|---|---|---|---|---|---|---|---|---|---|---|---|---|---|---|---|---|---|---|---|---|---|---|---|---|---|---|---|---|---|---|---|---|---|---|---|---|---|---|---|---|---|---|---|---|---|---|---|---|---|---|---|---|---|---|
|
Magnetic Resonance Imaging and Magnetic Resonance Angiography
MRI is the noninvasive imaging modality of choice for diagnosing and characterizing pediatric vascular anomalies (3,8,10). In comparison to ultrasound, MRI provides superior soft tissue differentiation and better defines positional relationships. In comparison to CT, MRI has three important advantages. It allows assessment of flow dynamics for distinguishing low-flow from high-flow lesions. It provides superior soft tissue characterization, and it uses no ionizing radiation.
Noninvasive MRA is being used with increasing frequency to map out feeding arteries and draining veins in vascular lesions that require sclerotherapy, transcatheter embolization, or surgical resection. MR angiograms rival conventional catheter angiograms in quality and are relatively noninvasive. They are performed at the same time as standard diagnostic MRI, are typically achieved without additional sedation, and, in contrast to CTA and conventional angiography, do not use ionizing radiation. 3D contrast-enhanced (CE) MRA can be repeated multiple times during arterial and venous phases of enhancement, providing time-resolved images without cumulative radiation exposure. Accordingly, MRA has a distinct safety advantage over other angiographic techniques for pediatric imaging (13). In addition, it has greater sensitivity than CTA and conventional angiography for low-flow vascular malformations.
During assessment of pediatric vascular anomalies, particularly those associated with specific syndromes, MRI and MRA are valuable tools for defining related abnormalities. For example, in patients with low-flow vascular malformations of an extremity, magnetic resonance venography (MRV) may be used to search for concomitant abnormalities of the superficial and deep venous systems (Klippel-Trenaunay syndrome [KTS]) (3). Similarly, in patients with suspected PHACES (anomalies of the Posterior fossa, facial Hemangiomas, cervical and cerebral Arteries, Cardiovascular system, Eye and ear, and Sternum) syndrome, MRI and MRA provide information about facial hemangiomas as well as anomalies of the posterior fossa, heart, aorta, and head and neck arteries (14).
The primary disadvantages of MRI and MRA are that they may be lengthy, noisy, and potentially frightening examinations for children. Special measures must be taken to reduce motion and eliminate anxiety during scanning. Technical parameters must also be modified to accommodate pediatric patients. Rapid scanning techniques should be used whenever possible.
Equipment
MRI scanners have undergone considerable development over the past decade. Improved gradient performance has reduced scan time, making vascular and soft tissue imaging much easier to perform in infants and children. Although techniques reported in this chapter are optimized for 1.5 T MRI scanners, the U.S. Food and Drug Administration (FDA) has recently approved use of field strengths up to 4 T for clinical imaging. To date, experience with high-field-strength MRI in children is limited, but benefits are likely to include improved signal-to-noise ratio (SNR), increased spatial and contrast resolution, better delineation of small vascular and soft tissue abnormalities, reduced scan duration, and lower contrast doses (15,16,17). In order to maximize application of these benefits to the pediatric population, concomitant advances in support equipment will be necessary. At this time, commercial MRI-compatible pulse oximeters and power injectors are available for high-field-strengths magnets, while MRI-compatible anesthesia infusion pumps and ventilators are only marketed for use in field strengths up to 1.5 T.
Sedation
The vast majority of children younger than 6 years of age require sedation for MRI. On occasion, even older children may benefit from sedation, particularly if they are developmentally delayed or if imaging protocols are long in duration. Sedation is more often needed for MRI than for CT due to longer scan duration, noisier image acquisition, and more intimidating gantry configuration (18).
To avoid complications of sedation, such as aspiration, respiratory arrest, hypoxic-ischemic brain injury, and death, safeguards must be implemented (18,19). Both the American Academy of Pediatrics and the American Society of Anesthesiologists have published guidelines recommending development and implementation of a structured sedation program by every institution at which pediatric sedation is performed (20,21). While prescribing sedation according to such guidelines is relatively safe and effective, sedating children without a structured policy may have disastrous consequences for the patient (19,22,23,24,25,26). Each institutional
sedation program should address variables that influence the safety and efficacy of pediatric sedation, including education and availability of personnel, patient screening, preparation and monitoring practices, sedative prescription, and complication preparedness. Only experienced persons who are capable of providing pediatric life support should administer sedation. Pediatric resuscitation equipment must be immediately available, and patients should be carefully chosen, prepared, and monitored (18).
sedation program should address variables that influence the safety and efficacy of pediatric sedation, including education and availability of personnel, patient screening, preparation and monitoring practices, sedative prescription, and complication preparedness. Only experienced persons who are capable of providing pediatric life support should administer sedation. Pediatric resuscitation equipment must be immediately available, and patients should be carefully chosen, prepared, and monitored (18).
Because patient visibility is limited in the MRI scanner, continuous electronic monitoring of sedated children is particularly important during scanning. MRI-compatible pulse oximeters with pediatric probes should be used with appropriate precautions to prevent thermal injury. MRI-compatible electrocardiographic equipment, blood pressure monitoring devices, and ventilators are commercially available as well and may be used when clinically indicated.
Certain patients with vascular anomalies—including those with mass lesions adjacent to the airway and those with systemic hemodynamic compromise—require special consideration when undergoing sedation for imaging procedures. Because endotracheal intubation, assisted ventilation, and pharmacologic support may be necessary, sedation is best performed in such cases by an anesthesiologist or other specialist experienced in advanced pediatric airway and circulatory management. Whenever possible, unstable respiratory or hemodynamic status should be corrected before the patient is transported to the imaging department.
A number of different sedatives are effective for pediatric imaging. Oral chloral hydrate (50 to 100 mg/kg; maximum dose 200 mg) is the most commonly used sedative in infants under 18 months of age. In children 18 months of age or older, intravenous sodium pentobarbital is the most widely used agent (2 to 6 mg/kg titrated slowly to effect; maximum dose 200 mg in children weighing <55 kg or 300 mg in children weighing >55 kg). A detailed review of safe sedation practices and available sedatives can be found in recent review articles (18,27).
Respiratory Motion
Short repetition time (TR) and echo time (TE) sequences that can be completed during a single breath hold negate the adverse effects of respiratory motion on MR image quality and are useful when imaging cooperative patients who have lesions in the chest or upper abdomen. When imaging uncooperative or sedated children who are unable to hold their breath, other techniques must be implemented to compensate for respiratory motion. The most commonly used technique is signal averaging, by which each phase-encoding step is repeated a number of times. The phase-encoding data are averaged, improving the SNR and reducing motion artifact. The disadvantage of this technique is that imaging time increases in direct proportion to the number of signals averaged (28).
Respiratory gating techniques have also been developed to reduce respiratory motion artifact. Respiratory gating synchronizes signal readout to occur at a consistent phase of the respiratory cycle, usually end-expiration, when motion is at a minimum. In patients with regular respiratory rates, images with sharper anatomic detail and reduced ghosting artifact are produced, but imaging time is increased because data acquisition is limited to a portion of the respiratory cycle. This technique is used predominantly with T2-weighted fast spin echo (FSE) sequences and is not compatible with T1-weighted imaging (29).
With respiratory ordered phase encoding (ROPE), phase-encoding steps are reordered according to the respiratory cycle, based on the patient’s breathing pattern at the beginning of the examination. Without increasing imaging time, ROPE minimizes ghosting artifact but does not eliminate image blurring (28,30). It is incompatible with FSE sequences due to the phase-ordering requirements of those sequences (31).
Clinical Protocols
Sequences commonly used for evaluating pediatric vascular anomalies are listed in Table 23-3. MRI protocols commonly begin with a bright blood gradient recalled echo (GRE) sequence to detect high-flow vessels. Presence of high-flow vessels distinguishes AVMs and hemangiomas from other pediatric vascular anomalies. For characterizing soft tissue content and defining lesion extent, T1-weighted spin echo (SE) sequences (with and without intravenous contrast) and T2-weighted FSE sequences are used. Both T1-weighted CE SE and T2-weighted FSE sequences are performed with frequency-selective fat saturation to increase conspicuity of lesions relative to fat.
When imaging hepatic hemangiomas and hemangioendotheliomas, dynamic CE MRI scanning is desirable for demonstrating characteristic early peripheral puddling of contrast and delayed central enhancement. Rapid T1-weighted gradient-echo images are obtained either throughout the lesion or at a single location at 20- to 60-second intervals for up to 10 minutes (32,33).
Because pediatric vascular anomalies may be larger than is apparent on physical examination, imaging should begin with a large field of view (FOV). Once the boundaries of a vascular lesion have been defined, application of a narrow FOV will increase spatial resolution. Reducing slice thickness also improves spatial resolution. Thicknesses of 3 to 6 mm are commonly used in infants and children.
Reducing slice thickness has the disadvantage of prolonging scan duration, which may result indirectly in patients awakening or moving before completion of all desired sequences. Accordingly, the need for increased spatial resolution should be carefully weighed and, if possible, counterbalanced by use of rapid imaging sequences and streamlined imaging protocols.
Reducing slice thickness has the disadvantage of prolonging scan duration, which may result indirectly in patients awakening or moving before completion of all desired sequences. Accordingly, the need for increased spatial resolution should be carefully weighed and, if possible, counterbalanced by use of rapid imaging sequences and streamlined imaging protocols.
Table 23-3 Magnetic Resonance Imaging and Magnetic Resonance Angiography of Pediatric Vascular Anomalies | ||||||||||||||
---|---|---|---|---|---|---|---|---|---|---|---|---|---|---|
|
The acquisition matrix (referring to the number of frequency-encoding steps × phase-encoding steps) has a direct relationship on both spatial resolution and scan duration. A 256 × 192 matrix is used for most SE sequences. When imaging small body parts or when using a large FOV, increased matrix size (up to 512 × 384) may be necessary to preserve resolution (28).
Although reducing FOV, reducing slice thickness, and increasing the acquisition matrix will improve spatial resolution in pediatric MRI, it also reduces the SNR. In order to maintain an adequate SNR, surface coils must be used. Very few surface coils have been designed specifically for imaging children. Therefore, creative use of surface coils designed for adults is necessary. Infants can be placed in standard adult head surface coils to image any body part. Older children are scanned with adult extremity, torso, or other surface coils. The smallest available surface coil that will cover the region of interest should be used. Especially when patients are sedated and unable to communicate, meticulous attention must be paid to manufacturer-recommended safety precautions in order to prevent serious thermal injuries.
When formulating MRI protocols, radiologists should keep in mind that scanning might be terminated prematurely if children awaken from sedation or are unable to be still. Imaging sequences that are critical to diagnosis should always be performed first, with additional sequences added only as time permits. A combination of sequences in at least two planes is used to maximize diagnostic yield in the available scan time. Familiarity with associated anomalies and characteristic patterns of disease distribution is required to ensure that a comprehensive diagnostic scan is completed.
MRA of pediatric vascular anomalies and associated syndromes may be performed in a number of ways. The most commonly used methods are time of flight (TOF), phase contrast (PC), and CE 3D MRA. With TOF angiography, contrast is achieved by subjecting stationary tissue to repeated radio frequency (RF) pulses until spins become saturated and give off relatively little signal. In comparison, fully magnetized spins entering the imaging volume, such as those in flowing blood, give off a large signal (34). Both two-dimensional (2D) and 3D TOF acquisitions are possible.
In 2D TOF, contiguous cross-sectional GRE images are generated in a plane perpendicular to the vessels of interest, usually the axial plane. By application of a spatial presaturation band proximal or distal to the imaging volume, either arterial or venous signal can be suppressed. Use of thin slices (on the order of 2 mm) reduces saturation of blood as it flows through the imaging plane. Flip angle and TR also affect the degree to which in-plane flow is saturated. Low flip angles (30 to 60 degrees) favor signal from slow-flowing blood, whereas high flip angles (60 to 90 degrees) favor signal from fast-flowing blood. At the same time, as the flip angle increases, unwanted signal from stationary tissue decreases. A flip angle of 60 degrees is often chosen in compromise (34). A long TR (>40 milliseconds) reduces saturation of in-plane flow but lengthens imaging time. A short TE reduces intravoxel dephasing, the phenomenon by which spins with different phases in the same voxel interfere with one another, resulting in signal loss (34).
Two-dimensional TOF MRA is degraded by presence of short T1 intravascular thrombus, which may mimic or obscure flowing blood. Due to in-plane saturation effects, the technique is potentially insensitive to slowly flowing blood and to vessels coursing parallel to the imaging plane. In addition to intravoxel dephasing, 2D TOF MRA is susceptible to ghosting artifacts from respiration and pulsatile flow (34).
For 3D TOF MRA, an RF pulse is applied to the entire imaging volume, rather than to individual slices, with every TR. The data are then segmented into individual slices by Fourier transformation. Because SNR depends on data from the entire imaging volume rather than from individual slices, SNR is greater for 3D than for 2D TOF. Three-dimensional imaging also permits smaller voxel dimensions, yielding isotropic spatial resolution and reduced intravoxel dephasing. The major disadvantage of 3D TOF imaging is that blood becomes progressively saturated as it flows through the larger imaging volume, resulting in signal variance along the length of a vessel. To diminish this effect, flip angle is reduced to 30 to 40 degrees and, in some cases, is varied as a function of depth in the volume. Choosing a TE such that fat and water are out of phase (e.g., 2.3 or 6.8 milliseconds at 1.5 T) helps to suppress background signal in the setting of a smaller flip angle. TR is lengthened to increase T1 recovery (34).
In PC angiography, contrast is achieved by manipulating the phases of spinning protons such that those in flowing blood are different than those in stationary tissues. The result is that signal intensity is proportional to flow velocity. Unlike TOF angiography, PC techniques can be used to quantify velocity and flow, to determine direction of flow, and to differentiate flowing blood from thrombus. PC angiography has excellent background suppression and can be sensitized to slow flow. Like TOF, it can be acquired in either 2D or 3D modes (34).
Two-dimensional PC imaging is performed in thick slabs rather than thin slices and requires a very short scan time. It is susceptible to intravoxel dephasing, and, unlike TOF techniques, does not provide cross-sectional source data perpendicular to the vessels of interest. Nor does it allow reprojection of angiographic images from any angle (34).
When performing PC angiography, one must specify a velocity-encoding value (Venc). PC images have the greatest sensitivity to blood flowing at velocities close to the Venc (34). When imaging pediatric vascular anomalies, it is sometimes useful to repeat rapid 2D PC acquisitions with different Venc values. Images performed with a low Venc (10 to 20 cm/second) are relatively sensitive to slow-flow and draining veins, whereas images performed with a high Venc (100 cm/second) better demonstrate high-flow lesions and feeding arteries (34).
The acquisition principles for 3D PC angiography are similar to those for 3D TOF. Data for the entire imaging volume are acquired with every TR and then segmented into individual slices by Fourier transformation. Three-dimensional PC imaging takes longer to perform than either 2D PC or 3D TOF imaging. Advantages include sensitivity to slow flow, high SNR, and good background suppression. Three-dimensional PC imaging has better spatial resolution and less susceptibility to intravoxel dephasing than 2D PC imaging. It is more susceptible to dephasing than 3D TOF. Postprocessing allows angular reprojection of angiographic images (34).
CE MRA has become the noninvasive angiographic method of choice for imaging many vascular anomalies. A T1-shortening agent is injected intravenously, and rapid 3D T1-weighted spoiled gradient echo (SPGR) imaging is performed during enhancement of the target vasculature. Gradient echo pulse sequences allow rapid data acquisition. Spoiling of residual magnetization after each echo improves T1 contrast and suppresses background signal. Because CE MRA does not rely on flow and phase-shift effects for intravascular contrast, motion and flow artifacts associated with TOF and PC imaging are not encountered (13).
Data for 3D CE MRA are collected over the entire scan time, resulting in excellent SNR. Isotropic spatial resolution can be achieved with submillimeter section thicknesses. Generally, a TE close to 2.3 milliseconds is used. This TE improves background suppression at 1.5 T, since signals from fat and water are out of phase. In addition, it minimizes the effects of dephasing and T2* signal decay. To reduce scan time, TR is shortened as much as possible, preferably without broadening bandwidth above 32 kHz. Narrower bandwidths improve SNR. Flip angles of 45 to 60 degrees are favored for arterial phase imaging, high-contrast doses, and long TRs, while flip angles of 30 to 45 degrees are reserved for venous phase imaging, lower contrast doses, and ultrashort TRs (<5 milliseconds) (13).
With 3D CE MRA, large FOV imaging can be performed in the plane of the vessels of interest, reducing both the number of slices and the amount of time necessary to image a large vascular territory. Partial Fourier imaging reduces scan time further by decreasing the number of phase-encoding steps without diminishing image resolution (13). On MRI scanners with high-performance gradients, imaging can be completed in seconds and, in patients who are old enough to cooperate, within a single breath hold.
Although many techniques have been described for CE MRA, time-resolved 3D MR digital subtraction angiography
(DSA) is best suited for evaluating pediatric vascular anomalies. With time-resolved 3D MR DSA, very rapid scanning allows acquisition of complete data sets before arrival of contrast in the target vessels as well as during arterial, parenchymal, and venous phases of enhancement. Digital subtraction is then performed to separate the vascular phases and improve the contrast-to-noise ratio (13). Patterns of contrast enhancement can be evaluated in addition to relative rates of enhancement in feeding arteries and draining veins.
(DSA) is best suited for evaluating pediatric vascular anomalies. With time-resolved 3D MR DSA, very rapid scanning allows acquisition of complete data sets before arrival of contrast in the target vessels as well as during arterial, parenchymal, and venous phases of enhancement. Digital subtraction is then performed to separate the vascular phases and improve the contrast-to-noise ratio (13). Patterns of contrast enhancement can be evaluated in addition to relative rates of enhancement in feeding arteries and draining veins.
A major benefit of time-resolved 3D MR DSA techniques in pediatric imaging is that precise bolus timing is unnecessary. Contrast is injected rapidly, usually at a rate of 2 to 3 mL/second, and serial acquisitions of less than or equal to 10-second duration are performed in rapid succession. At least one acquisition will certainly coincide with the arterial phase of enhancement, and at least one will coincide with the venous phase of enhancement. Accordingly, there is no need for use of bolus-tracking techniques or test injections to determine contrast arrival time in the target vasculature (13).
For optimal isolation of arterial and venous phases in children with rapid recirculation times, temporal resolution must be maximized. Most high-performance MRI scanners are now capable of completing a 3D MRA acquisition in 6 to 10 seconds. Data processing techniques have been developed to improve temporal resolution further. 3D TRICKS (3D time-resolved imaging on contrast kinetics) is one such method. It uses frequent central k-space sampling, zero-filling, and temporal interpolation of data to generate 3D images as frequently as every 2 seconds (13).
For targeted 3D CE MRV of an extremity, direct venography has also been described (35). Peripheral intravenous access is secured in the affected extremity, and contrast is injected directly into the venous system during imaging. A relatively high-contrast dose is used (0.3 mmol gadolinium chelate/kg), diluted at least 10:1 with normal saline to prevent signal loss from T2 shortening and to allow prolonged injection. 3D SPGR imaging is begun soon after initiation of contrast injection and repeated several times until the venous system is completely filled. Digital subtraction of a precontrast scan improves results. This technique is superior to indirect CE MRV in terms of contrast-to-noise ratios (35).
Intravenous Contrast
When administered intravenously, gadolinium chelates shorten T1 relaxation times, resulting in positive contrast enhancement (increased signal intensity) on T1-weighted pulse sequences. After injection, they distribute predominantly in the blood pool and extracellular fluid compartments of the body, where they remain until they are excreted by the kidneys. Redistribution from the blood pool to the extracellular fluid space is completed in approximately 11 minutes (13). Biologic half-lives of the contrast agents are between 1 and 2 hours (36).
With respect to MRI of pediatric vascular anomalies, intravenous contrast agents help to distinguish lymphatic malformations—which enhance only peripherally or not at all—from enhancing hemangiomas and venous malformations. For standard CE MRI at 1.5 T, gadolinium chelates are generally injected at a dose of 0.1 mmol/kg. At 3 T, the contrast dose may be halved (0.05 mmol/kg) because of increases in SNR and contrast resolution. For CE MRA, doses of 0.2 to 0.3 mmol/kg are used (35,37).
Intravenous contrast may be injected either manually or with an MRI-compatible power injector. In comparison to manual injection, power injection provides more uniform delivery of contrast material with no delay between injection of the contrast agent and saline flush (37). Power injection is used predominantly for CE MRA and is not necessary for standard CE MRI. It must be used with caution in infants and children who are sedated or unable to communicate, particularly when injection is performed through intravenous catheters located in hands, wrists, or feet. Regardless of the mode of injection, intravenous positioning of the catheter with both free blood return and unrestricted saline injection should be confirmed prior to contrast administration.
Contrast injection is most often performed through 20 to 24 gauge peripheral intravenous catheters. Either manual or power injection can be performed via this route. Alternatively, contrast may be injected by hand through central venous catheters or peripherally inserted central catheters (PICCs). Power injection of gadolinium contrast agents through central lines and PICCs has not been studied rigorously in children.
Risks associated with extravasation are lower for gadolinium chelates than for iodinated CT contrast agents because of the lower osmolality and lower volumes of contrast used for MRI (13,38). Nonetheless, animal models demonstrate that extravascular gadolinium chelate extravasation may lead to soft tissue inflammation and necrosis. Soft tissue damage is greatest with gadopentetate dimeglumine (Magnevist) and least with gadoteridol (ProHance) and gadodiamide (Omniscan). These latter agents are lower in osmolality (39).
When possible, injection sites are monitored visually so that contrast extravasation can be recognized and stopped
immediately. Visual inspection is most important when patients are nonverbal or sedated and are unable to report injection site pain. Monitoring the injection site is generally not problematic for standard CE MRI, because the patient may be removed from the bore of the magnet prior to contrast injection and then repositioned in time to begin scanning. Although scanning is usually begun within 1 minute of contrast injection, precise bolus timing for standard CE MRI sequences is unnecessary.
immediately. Visual inspection is most important when patients are nonverbal or sedated and are unable to report injection site pain. Monitoring the injection site is generally not problematic for standard CE MRI, because the patient may be removed from the bore of the magnet prior to contrast injection and then repositioned in time to begin scanning. Although scanning is usually begun within 1 minute of contrast injection, precise bolus timing for standard CE MRI sequences is unnecessary.
For dynamic CE MRI or CE MRA, the patient must be positioned within the magnet bore to allow initiation of scanning during or immediately after contrast injection. Contrast is generally injected through a length of tubing, and the injection site may not be visible from outside the magnet bore. In this setting, power injection of the contrast agent and saline flush must be performed with caution.
Contrast injection should always be followed by an intravenous saline flush. The volume of gadolinium agents used in pediatric MRI is very small, and a large percentage may remain in the intravenous catheter tubing after injection. A saline flush clears contrast from the tubing. It prevents artifactual signal loss due to intravenous pooling of concentrated gadolinium (40). In addition, it reduces the risk of secondary venous thrombosis (13). Intravenous access should be maintained for at least 10 minutes following contrast injection so that intravenous fluids and medications can be administered, if needed, for allergic reaction. Serious allergic reactions are rare (36).
Computed Tomography and Computed Tomographic Angiography
Although MRI is preferred for assessing pediatric vascular anomalies, CE CT may replace or complement MRI in some cases. While MRI takes 20 minutes or more to perform, CT can be completed in less than a minute and provides a rapid alternative to MRI in children with unstable cardiovascular status or respiratory compromise. It is useful in patients who have contraindications to sedation or MRI. In addition, it has a role in imaging patients with embolization coils or surgical clips in whom MRI is degraded by magnetic susceptibility artifact.
In the setting of pediatric vascular anomalies, the primary roles of CT are to characterize soft tissue content; to define positional relationships; and, in some cases, to delineate osseous involvement. CE CT is preferable to MRI in imaging vascular anomalies of the neck, bowel, and lungs. Although CT is better than MRI for demonstrating phleboliths in venous malformations, plain radiographs reveal phleboliths at a lower radiation dose to the patient (3).
High-resolution CTA has become feasible in recent years with introduction of helical and multislice CT scanners. Large vascular territories can now be imaged within seconds, minimizing the detrimental effect of motion on image quality. CTA has better spatial resolution than MRA but uses ionizing radiation. Radiation exposure is cumulative, and doses are particularly high when multiphasic scans are performed to resolve arterial and venous phases of enhancement. At this time, concerns regarding the potential for carcinogenesis in infants and children limit the use of multiphasic CTA in the pediatric population. It should be used only when imperative for diagnosis and when MRA is contraindicated.
Examples of specific imaging protocols are included in Table 23-4. When performing CT and CTA in children, unique technical considerations arise, stemming from the wide spectrum of patient sizes, hemodynamic states, and maturity levels encountered.
Equipment
Since 1990, CT technology has been rapidly and continually improving. Multislice scanners, collecting several channels of data simultaneously, provide a speed advantage over older single-slice helical and incremental models. Subsecond
gantry rotation times also increase scan speed. Radiologists can use increased speed to shorten scan duration (scanning quickly through a region of interest without reducing collimation) or to increase z-axis resolution (scanning for a given period of time with thinner collimation). When imaging pediatric vascular anomalies, increased speed translates into reduced patient motion, improved 2D and 3D image quality, and greater latitude with respect to scan timing and contrast injection.
gantry rotation times also increase scan speed. Radiologists can use increased speed to shorten scan duration (scanning quickly through a region of interest without reducing collimation) or to increase z-axis resolution (scanning for a given period of time with thinner collimation). When imaging pediatric vascular anomalies, increased speed translates into reduced patient motion, improved 2D and 3D image quality, and greater latitude with respect to scan timing and contrast injection.
Table 23-4 Sample Computed Tomography and Computed Tomographic Angiography Protocols for Evaluation of Pediatric Vascular Anomalies | ||||||||||||||||||||||||||||||||||||||||||||||
---|---|---|---|---|---|---|---|---|---|---|---|---|---|---|---|---|---|---|---|---|---|---|---|---|---|---|---|---|---|---|---|---|---|---|---|---|---|---|---|---|---|---|---|---|---|---|
|
Image processing workstations, particularly those with intuitive user interfaces, automated operations, and ultrafast performance characteristics, facilitate rapid creation of multiplanar and 3D images, thereby improving scan interpretation, treatment planning, and communication of results. Picture archival and communications systems (PACS) also ease handling of the massive amounts of data that result from high-resolution vascular CT and CTA.
Sedation
To eliminate misregistration artifact in CT, patients must remain motionless during image acquisition. Neonates and infants under 12 months of age, when fed and swaddled, may sleep through a CT examination. Older children sometimes require sedation, although its use has diminished considerably in recent years with the use of faster helical and multislice CT scanners (41,42,43). Pappas et al. (43) reported a sedation rate of only 3.3% in children younger than 7 years of age who were undergoing multislice body CT (43). In practice, strict age restrictions for sedation are not useful. Rather, the decision to give sedation or not should be adapted to the needs of the individual child (18). The same sedation safety precautions should be used for CT as for MRI.
Respiratory Motion
Misregistration due to respiratory motion may diminish the diagnostic value of CT directed to the chest or abdomen. This is particularly true when target lesions are small relative to respiratory excursion and when respiratory rates are rapid, as is often the case in children. Whenever possible, respiratory motion should be minimized during image acquisition. Cooperative children over 5 to 7 years of age are able to suspend respiration voluntarily for periods of up to 30 seconds, providing a satisfactory window in which to complete data acquisition (44). A practice session before scanning allows appraisal of each child’s breath holding skills and increases the likelihood that he or she will follow instructions correctly during imaging.
In children under 5 to 7 years of age who are too young to hold their breath voluntarily, images obtained during quiet respiration may be diagnostic. Ventilation can be suspended briefly in children who are intubated and paralyzed under general anesthesia. Alternatively, a 15- to 25-second respiratory pause (Hering-Breuer reflex) may be induced in children who are sedated but not intubated by using positive pressure ventilation applied through a face mask apparatus (45). This latter technique, known as controlled ventilation, is noninvasive and well tolerated by both infants and children. It has been applied primarily to high-resolution CT scanning of the chest but is also applicable to other pediatric CT protocols. Two operators perform the maneuver, one controlling the face mask device and the second applying gentle cricoid pressure to minimize air passage down the esophagus. Through the face mask, positive pressure (25 cm water) is applied to augment the patient’s spontaneous inspiratory efforts. Additional breaths are delivered to induce hypocarbia and apnea. For a given patient, the number of additional breaths required to induce apnea as well as the duration of the respiratory pause are reproducible and can be determined by a “trial run” before CT scanning. Once apnea is achieved, maintenance of positive pressure lengthens its duration and allows chest imaging during full lung inflation (45).
Radiation Dose, mA, kVp, and Pitch
Children are more sensitive than adults to radiation and have a higher risk of experiencing the carcinogenic and genetic effects (46). In addition, because x-ray beam attenuation decreases as body mass decreases, CT imparts a higher effective radiation dose to children than to adults (47). Based on models assuming a linear extrapolation of risks from intermediate to low doses, Brenner et al. (48) have estimated that the lifetime cancer mortality risk attributable to radiation exposure at the age of 1 year is 0.18% for abdominal CT and 0.07% for head CT (48). Although the veracity of these risk estimates is debated, radiologists widely agree that patient radiation exposure should be kept as low as is reasonably achievable (ALARA) (49,50). To this end, care should be taken to avoid unnecessary scans, to implement radiation-reducing technology, and to choose age-appropriate scan parameters. When feasible, CT should be replaced by other diagnostic imaging modalities that do not use ionizing radiation, such as ultrasound and MRI. It is also important that radiologists and technologists be educated about specific parameters that affect radiation dose and take an active role in minimizing exposure to each patient.
The first parameter to consider is tube current, often expressed as a function of time (milliamperes-seconds or mAs). mAs determines the quantity of x-rays to which the patient is exposed and is directly related to radiation dose. The number of x-rays required to create a diagnostic image
decreases as patient size decreases, because small patients attenuate the x-ray beam less than large patients. Therefore, pediatric CT can and should be performed with a lower mAs than adult CT (49). A number of strategies have been proposed to tailor mAs to patient size. The most commonly used strategies involve mAs gradation according to patient weight, height, or cross-sectional dimensions (49,51,52). Specific recommendations regarding mAs are included in Table 23-4.
decreases as patient size decreases, because small patients attenuate the x-ray beam less than large patients. Therefore, pediatric CT can and should be performed with a lower mAs than adult CT (49). A number of strategies have been proposed to tailor mAs to patient size. The most commonly used strategies involve mAs gradation according to patient weight, height, or cross-sectional dimensions (49,51,52). Specific recommendations regarding mAs are included in Table 23-4.
The potential disadvantage of decreasing mAs is increased image noise. Noise is inversely related to the number of x-rays that reach the CT detectors. While reducing mAs in a given patient increases image noise, this effect is balanced as patient size decreases by a reduction in x-ray beam attenuation (49). Accordingly, mAs can be reduced in smaller patients without degradation of image quality, assuming no change in section thickness. If section thickness is reduced to improve spatial resolution in small patients, there will be a concomitant increase in image noise. Section thickness, mAs, and noise must be balanced to achieve diagnostic image quality while minimizing radiation dose.
Several authors suggest that CT tube potential can also be reduced in small patients without significant degradation of image quality (47,53,54,55). Tube potential determines x-ray beam energy and is exponentially related to radiation dose (46,52). Most CT examinations are performed with a tube potential of 120 peak kilovoltage (kVp) (56). Theoretically, lowering tube potential to 80 kVp would improve bone, iodine, and soft tissue contrast in small patients while reducing radiation dose as much as 65% (47,54,57). Because of the complex relationship between tube potential, image noise, tissue contrast, and patient size, additional study into modulation of this parameter is needed.
Pitch is a third parameter that can be adjusted to reduce radiation dose in helical CT. Increasing pitch reduces redundant tissue exposure and shortens the time during which the x-ray beam is turned on. Few comparisons of pitch and radiation exposure have been made in children. Vade et al. (58) reported a 33% reduction, and Donnelly et al. (49) reported a 28% reduction in radiation dose using a pitch of 1.5 as compared to 1.0 on single-slice helical scanners. To maintain constant image noise levels, some multislice CT scanners automatically increase tube current (mA) in proportion to increases in pitch (59). On these scanners, the benefits of increasing pitch are negated unless mA is manually reduced. The potential disadvantage of increasing pitch is broadening of the section sensitivity profile with loss of z-axis resolution. Reducing collimation and using an overlapping image reconstruction interval minimizes this effect (60). Helical CT and CTA in children are most often performed with a pitch of 1.5 (56). When subsecond gantry rotation intervals are used, a pitch close to 1.0 is advised.
In comparison to single-slice CT, multislice CT geometry changes radiation dose in several ways (61,62). Septa between detectors in the z-axis direction absorb radiation that consequently contributes to patient dose but not to image data. This effect increases as the number of detector rows increases. Radiation dose is also increased by broadening of the x-ray beam in the z-axis direction, such that edges of the beam create a penumbra beyond the margins of the active detector elements. While ensuring uniform x-ray exposure to each detector element, broadening of the x-ray beam further reduces radiation dose efficiency (61). At the same time, dose efficiency is somewhat increased because beam collimation is relatively large with respect to focal spot size (high umbra:penumbra ratio) (62).
Growing concern regarding CT’s potential for carcinogenesis has led CT manufacturers to implement both hardware and software modifications that reduce radiation dose (51,54,63,64). Positive changes include implementation of solid-state detectors, improved anode ratings, focal spot tracking, and soft-beam x-ray filtration (50,52,65). Commercial CT scanners now allow preprogramming of pediatric-specific tube current settings for a number of standard imaging protocols (63,64). Real-time current modulation has also been developed, tailoring mA in response to changes in patient geometry and x-ray absorption during data acquisition (52,66). In addition, commercial CT consoles are now required to display the volume CT dose index (CTDIvol) (52). CTDIvol estimates the average radiation dose in a scan volume with reference to a standardized CT phantom. Some CT scanners also display the dose length product [DLP = CTDIvol (milligrays) × Scan length (cm)]. Access to these dose descriptors at the time of scan planning enables radiologists and technologists to predict and actively reduce radiation exposure on a case-by-case basis (46).
Additional patient protection is achieved by shielding radiosensitive organs (lenses, thyroid gland, breasts, and gonads) during CT scanning. Organs both inside and outside of the scan volume may be shielded (52). Fricke et al. (67) reported a 29% reduction in breast radiation dose with bismuth in-plane shielding during multislice CT of the chest. Similarly, Hopper et al. (68) reported a 40% to 50% reduction in radiation dose to the lenses with bismuth-coated latex eye shielding during CT of the head. Both groups claimed no loss of diagnostic information. Beaconsfield et al. (69) demonstrated a 45% reduction in thyroid radiation and a 76% reduction in breast radiation with lead shielding of these organs during CT of the head. Hidajat et al. (70)
showed a 95% reduction in absorbed testicular dose with use of a testis shield during abdominal CT.
showed a 95% reduction in absorbed testicular dose with use of a testis shield during abdominal CT.
Additional Scan Parameters
Several additional scan parameters must be selected when planning CT scans of pediatric vascular anomalies. These include collimator thickness, section thickness, reconstruction interval, FOV, and z-axis coverage.
Collimator thickness is chosen before scanning is initiated. For conventional and single-slice helical CT, collimator thickness is equivalent to nominal section thickness. Values in the range of 3 to 5 mm would be appropriate for imaging pediatric vascular anomalies. Thinner collimation is generally avoided when imaging children with conventional or single-slice CT scanners, because an increase in mA is required to keep quantum noise levels constant. Consequently, CT angiograms, 3D reconstructions, and multiplanar reformations created on these scanners are of lower quality than those created on multislice CT scanners.
On multislice CT scanners, collimator thickness defines the minimum section thickness that can be achieved after scanning is completed. Values in the range of 1.0 to 2.5 mm would be appropriate for imaging pediatric vascular anomalies, as long as mAs is not increased concurrently. By a process known as z-filtering, section thickness can then be manipulated retrospectively. Sections of 3 to 5 mm with noise levels comparable to those achieved on conventional or single-slice scanners are created for axial image interpretation. Sections of 1.0 to 2.5 mm can also be generated to serve as superior source images for CTA, 3D reconstruction, and multiplanar reformation. Accordingly, the advantages of high-resolution, thin-collimation imaging can be achieved without penalty in terms of quantum noise or radiation dose. Choosing a reconstruction interval that is 33% to 50% smaller than the section thickness reduces partial volume averaging effects and also improves the quality of postprocessed images (44).
FOV should closely approximate the diameter of the anatomic region of interest. Because pixel size is directly proportional to FOV, decreasing FOV improves spatial resolution and reduces partial volume averaging effects (55). To prevent unnecessary radiation exposure, scan coverage in the z-axis direction must be closely confined to the anatomic region of interest. When determining FOV and z-axis coverage, radiologists should be aware that pediatric vascular anomalies may be larger than is apparent on physical examination, may be multifocal, and may be associated with other anomalies. FOV and z-axis coverage should accommodate potential patterns of disease distribution.
Frontal and/or lateral digital scout radiographs, used to determine FOV and z-axis coverage, are performed with a low tube current (30 to 40 mA). Precontrast axial CT scans are generally avoided unless imperative for diagnosis. The same is true of multiphase scans, which may double or triple radiation dose. If multiphase imaging is required, whether to evaluate arterial and venous anatomy or to assess contrast enhancement patterns over time, then it should be performed with a low mA and, when possible, at a single scan location.
Intravenous Contrast
Optimum contrast enhancement of pediatric vascular anomalies depends on predictable and uniform delivery of contrast material to the target organs and vessels. Injection techniques applied to infants and children are different than those used in adults. Smaller patients require smaller contrast doses; smaller intravenous catheters mandate reduced injection rates and altered scan delays. Because children come in a wide range of sizes, contrast enhancement techniques are scaled to the individual patient.
Use of low-osmolality nonionic intravenous contrast is preferred in pediatric CT, despite its increased cost (71). In comparison to ionic contrast media, the nonionic agents have fewer minor side effects such as unpleasant taste, nausea, vomiting, flushing, and injection site discomfort (72). Although not life-threatening, these side effects may lead to patient motion during image acquisition (72,73). Indeed, reduced patient motion and improved scan quality have been demonstrated with use of nonionic contrast agents in both children and adults (72,74).
Contrast doses ranging from 1 to 3 mL/kg are used for pediatric CT and CTA, with a dose of 2 mL/kg used most frequently (300 mg iodine/mL, total dose not to exceed 5 mL/kg) (56,60). To reduce perivenous artifact resulting from concentrated iodine, contrast may be diluted 3:1 with normal saline (44,75). Although both manual injection and power injection are used for pediatric CT and CTA, power injection provides more uniform and predictable enhancement. Its use is gaining acceptance but is not universal due to concerns regarding the potential for catheter rupture, contrast extravasation, and soft tissue injury (44,56,76
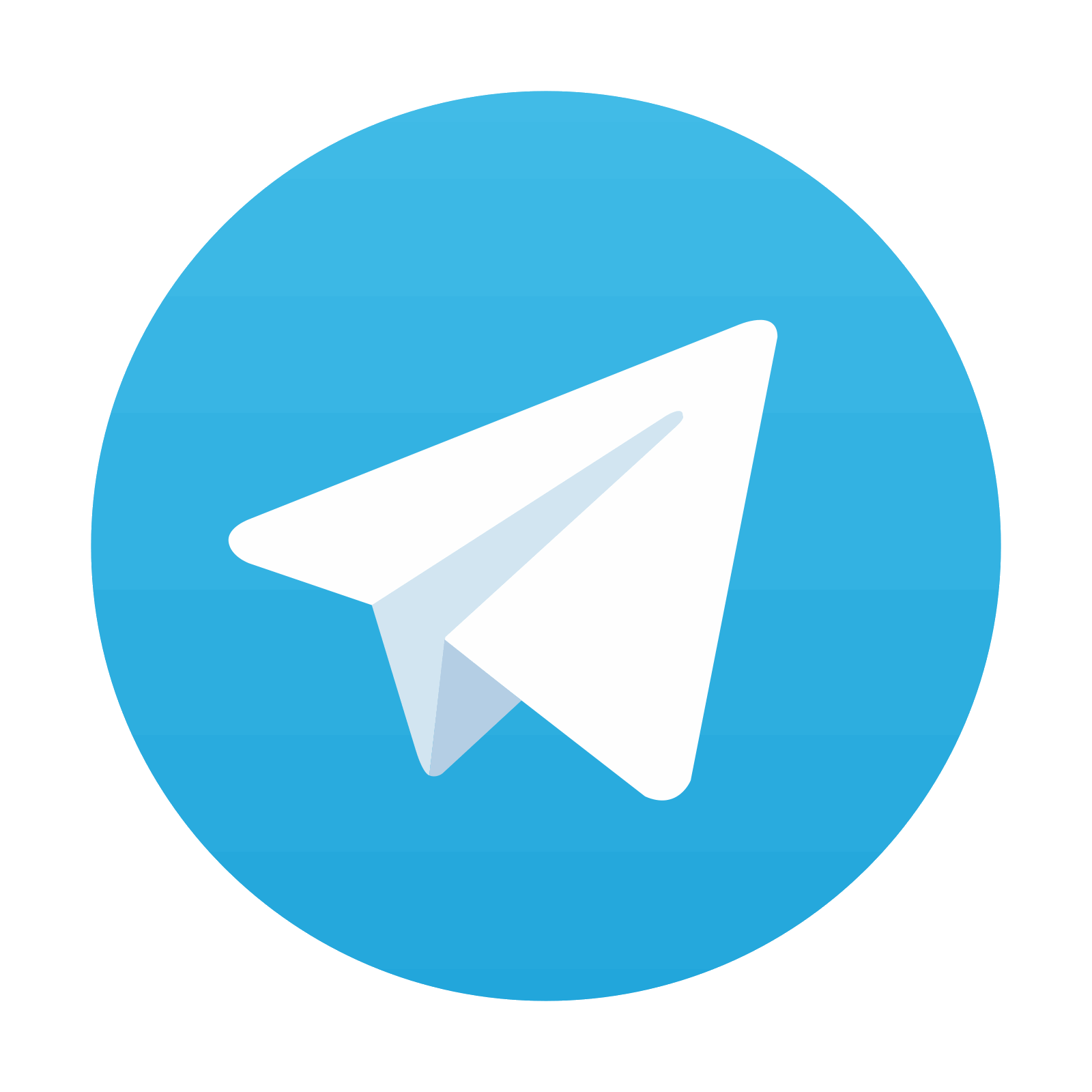
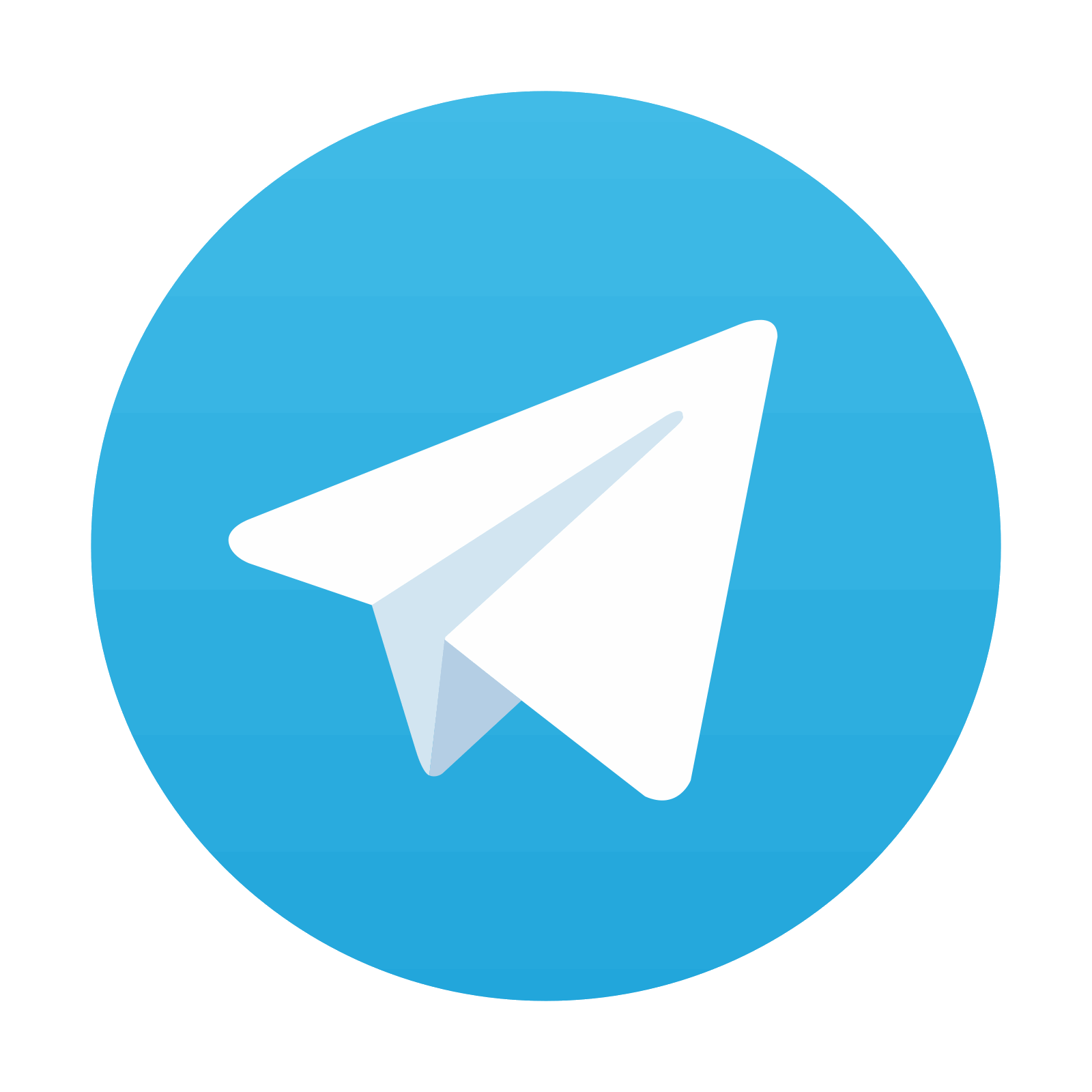
Stay updated, free articles. Join our Telegram channel
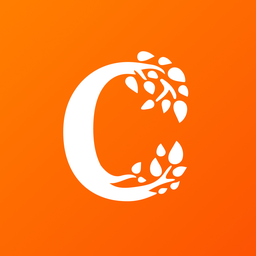
Full access? Get Clinical Tree
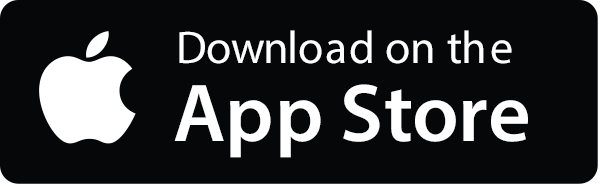
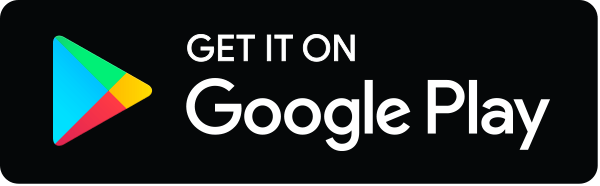
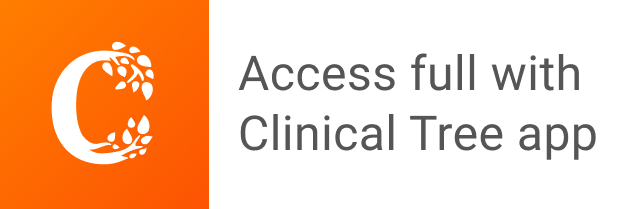