Doppler may be applied to determine the peak velocity in the region. Continuous-wave Doppler emits and listens simultaneously. Thus, continuous-wave Doppler will assess all velocities within a line of interrogation—velocities within as well as proximal to and distal to the site of interest. Combining pulsed-wave and continuous-wave Doppler when assessing disturbed flow patterns is ideal to give the location of the turbulence and the maximum velocity.
Table 72.1 Views and Sweeps for Echocardiographic Imaging of the Child with Suspected Congenital Heart Disease | ||||||||||||||||||||||||||||||||||||||||||||||||||||
---|---|---|---|---|---|---|---|---|---|---|---|---|---|---|---|---|---|---|---|---|---|---|---|---|---|---|---|---|---|---|---|---|---|---|---|---|---|---|---|---|---|---|---|---|---|---|---|---|---|---|---|---|
|
anomalous pulmonary venous connection (TAPVC). A left superior vena cava has no hemodynamic significance in the structurally normal heart. Typically, this vessel crosses anterior to the left pulmonary artery and enters the coronary sinus posteriorly. However, bilateral superior venae cavae are common in heterotaxy syndrome. Identification of this anomaly becomes important particularly if single-ventricle palliation is planned (Fig. 72.5). Without a bridging vein between the superior venae cavae, a bilateral bidirectional superior cavopulmonary anastomosis is necessary in single-ventricle palliation; when a large enough bridging vein is present, the left superior vena cava can be ligated rather than connected to the pulmonary artery. In heterotaxy syndrome, the left superior vena cava may empty directly into the left atrium or by way of an unroofed coronary sinus resulting in a right-to-left shunt.
![]() Fig. 72.3. Coronal view used to determine situs. Situs solitus or normal situs is seen with the aorta to the left of the spine and the inferior vena cava (IVC) running through the liver on the right. |
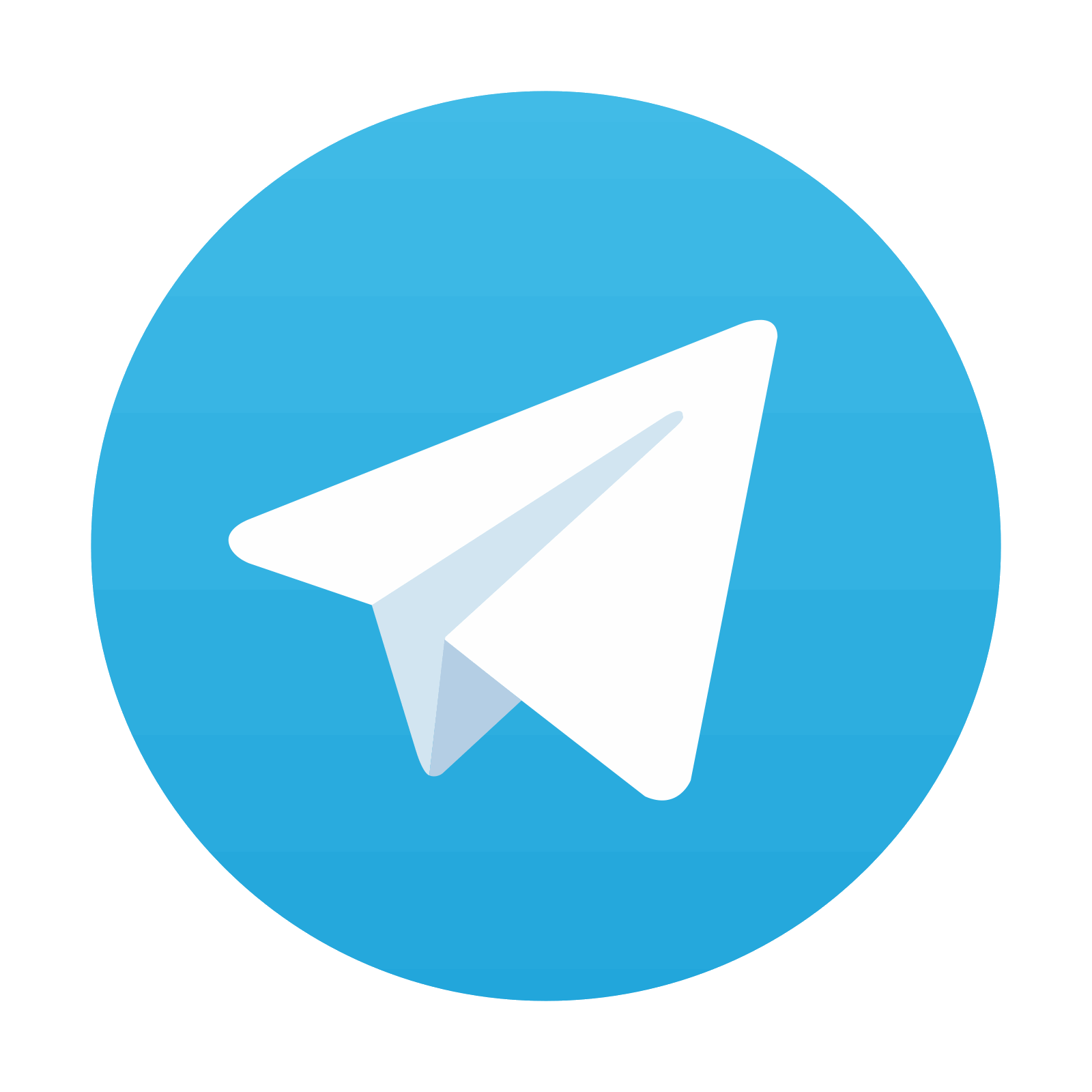
Stay updated, free articles. Join our Telegram channel
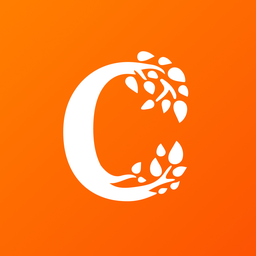
Full access? Get Clinical Tree
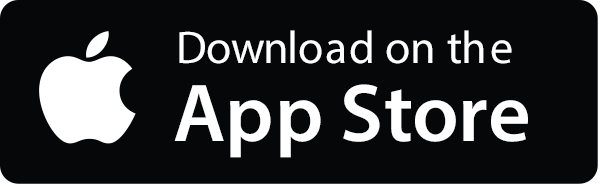
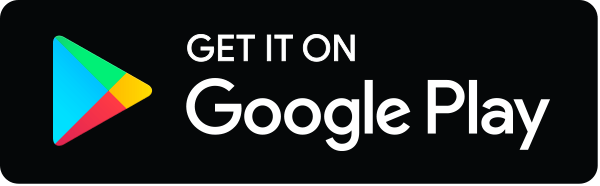