Contrast Medium Administration in Magnetic Resonance Angiography
Jeffrey H. Maki
Lee M. Mitsumori
The emergence of magnetic resonance angiography (MRA) as an accurate, noninvasive alternative to conventional catheter angiography has been largely due to the marriage of MR technological advances with MR contrast agents. MR angiography began in the mid 1980s (1), using noncontrast techniques such as time-of-flight (TOF) and phase contrast (PC) based on the motion of flowing blood. Formerly, these techniques were the mainstay of MRA and now are largely relegated to studying the central nervous system blood vasculature (2).
In 1988, the approval of the first MR contrast agent for clinical use in the United States (3) set the stage for exciting developments to come in the early 1990s with applications extending to MRA. The first contrast-enhanced MR angiograms (CE-MRA) required a hefty 5-minute-and-10-second acquisition time (4), and although image quality paled in comparison to what can be accomplished today, the results captured the attention of the MR community. Just over a decade later, CE-MRAs of astonishing quality can be produced in seconds, with the same class of MR contrast agent first used in central nervous system (CNS) magnetic resonance imaging (MRI) back in 1988! While MR technology continues to advance at remarkable rates with higher field strength (3 Tesla) clinical machines (5), improved coil technology (6), and parallel imaging
acceleration techniques (7), so too does MR contrast development.
acceleration techniques (7), so too does MR contrast development.
This chapter will broadly discuss MR contrast agents in the context of their association with MRA. Newer contrast agents are being developed and while some are already in use in Europe, availability is lagging in the U.S. Decisions regarding the acquisition technique, the choice of contrast media, and method of administration for a given agent will be necessary to optimally perform MRA as one seeks to best answer the clinical question at hand. This chapter attempts to provide tools to assist in such decisions.
First, a review of basic MR physics will enable the understanding of selected principles important for further understanding the mechanism of different classes of contrast agents as they apply to CE-MRA. Next, the physics and efficacy of contrast agents will be discussed in terms of (a) physical properties and mechanism of action for each agent, (b) the pharmacokinetic properties that govern the diagnostic efficacy of an agent at the desired site of action, and (c) the safety issues related to MR contrast agent use. Following this, details of contrast administration, including injection techniques; dosing and timing issues and how they relate to commonly seen CE-MRA artifacts; and specific MRA acquisition techniques will be covered. Finally, some brief comments regarding future direction of MR contrast agents and CE-MRA will be presented.
Before embarking on a comprehensive discussion of contrast agent principles, safety, and administration, it is worth emphasizing that MR contrast agents are considered drugs and as such are regulated by the U.S. Food and Drug Administration (FDA). When a new drug is submitted to the FDA for approval, the manufacturer presents well-controlled investigations supporting its labeling claims. Once that drug is approved, the manufacturer is only permitted to advertise the drug for approved indications and can only do so using the information approved by the FDA for use in labeling (8).
At present, none of the four traditional gadolinium extracellular contrast agents approved by the FDA (Table 5-1) include indications for MRA, and hence, the manufacturers cannot advertise their products in any way for use with MRA. This is in no way a statement about the safety or efficacy of these agents for MRA, but rather is a default position because the manufacturers did not present the FDA with data to specifically support a claim that their agent was evaluated in the context of MRA procedures. Thus, in the United States, administration of an MR contrast agent to perform MRA is considered “off-label” use.
Physicians are permitted by the FDA to lawfully deviate from the conditions for use as approved in the drug package insert as part of the “practice of medicine” (9), and such off-label uses have been upheld by the courts. While the FDA frowns at the marketing of off-label applications, it does permit off-label use provided that use occurs in an independent medical program—meaning a program either not supported by industry or, if partially supported, following specific rules regarding disclosure of commercial sponsors and maintaining control of content and speakers (8). Thus, the radiologist is ultimately responsible for selecting the contrast agent and dose for a particular indication.
Basic principles
One of the most unique and clinically exploitable properties of MRI is the rich variety of contrast mechanisms available. Indeed, MRI is well known for the rich intrinsic soft tissue contrast found in straightforward acquisitions emphasizing T1 or T2 weighting. The imaging features may be modified by the addition of exogenous contrast agents and how these agents are distributed.
In comparison to CT contrast agents, which function simply through their ability to scatter or absorb x-ray photons, MR contrast agents work indirectly by chang-ing the local magnetic environment of tissue, thereby modifying intrinsic relaxation rates of tissues. In order to more fully understand this process, it is necessary to briefly review the basic principles of how MR signals are generated.
Magnetic Resonance Basics
Nuclear magnetic resonance is a phenomenon exhibited by atoms having odd numbers of nucleons (protons and neutrons) (10). The most clinically relevant of these is the proton (1H), due to its high abundance given the approximate 70% water (H2O) content of the human body. Almost all clinical MRI is proton MRI. Other biologically pertinent atoms exhibiting the MR phenomena include 13C, 23Na, and 31P, although these species are much less abundant in the human body and currently are rarely used for clinical imaging.
The hydrogen nucleus, a single proton, can be considered a spinning positive charge, and thus, it generates a magnetic field. This is often described as a magnetic moment or dipole. When a population of protons is placed into a static magnetic field (called B0), each proton’s magnetic moment aligns either parallel or antiparallel to B0 (due to two discrete quantum mechanical energy states), with just a slightly greater fraction aligning parallel to B0 such that there is a small net magnetic moment parallel to B0.
TABLE 5.1 Physiochemical Properties and Approval Status of All Currently Approved and Selected Experimental MR Contrast Agents | |||||||||||||||||||||||||||||||||||||||||||||||||||||||||||||||||||||||||||||||||||||||||||||||||||||||||||||||||||||||||||||||||||||||||||||||||||||||||||||||||||||||||||||||||||||||||||||||||||||||||||||||||
---|---|---|---|---|---|---|---|---|---|---|---|---|---|---|---|---|---|---|---|---|---|---|---|---|---|---|---|---|---|---|---|---|---|---|---|---|---|---|---|---|---|---|---|---|---|---|---|---|---|---|---|---|---|---|---|---|---|---|---|---|---|---|---|---|---|---|---|---|---|---|---|---|---|---|---|---|---|---|---|---|---|---|---|---|---|---|---|---|---|---|---|---|---|---|---|---|---|---|---|---|---|---|---|---|---|---|---|---|---|---|---|---|---|---|---|---|---|---|---|---|---|---|---|---|---|---|---|---|---|---|---|---|---|---|---|---|---|---|---|---|---|---|---|---|---|---|---|---|---|---|---|---|---|---|---|---|---|---|---|---|---|---|---|---|---|---|---|---|---|---|---|---|---|---|---|---|---|---|---|---|---|---|---|---|---|---|---|---|---|---|---|---|---|---|---|---|---|---|---|---|---|---|---|---|---|---|---|---|---|
|
It is this net magnetic moment (sometimes called proton spin) that is manipulated to form the MR signal. Of note, the preferential parallel fraction is only 5 per million but does increase as B0 increases, providing one explanation why increased magnetic fields provide more signal.
The magnetic moment of a proton precesses around B0 at a frequency called the Larmor frequency. The Larmor frequency depends on the strength of the applied magnetic field B, with the angular frequency ω described as ω = γB, where γ/2π = 42.57 MHz/T is called the gyromagnetic ratio. MR signal is generated by applying radio frequency (RF) energy at the Larmor frequency (resonance), thereby tipping or “nutating” the magnetic moment away from the axis of B0. As the proton precesses around B0, it can be thought of as a magnet rotating within a magnetic field and as such will induce current to flow in a nearby conducting coil (the MR receiver coil) according to the Faraday law of electromagnetic induction (10). Current that is induced in the receiver coil is proportional to the transverse component of magnetization (i.e., the component perpendicular to the axis of B0). This current oscillates at the Larmor frequency and becomes the basis for creation of an MR image.
The property of how an individual spin “relaxes” to realign with B0 is described by the T1 relaxation time, or spin-lattice relaxation. Similarly, the properties of how a collection of spins (we cannot image just one!) precess at slightly different Larmor frequencies and hence lose signal due to phase cancellation is referred to as the T2, or spin-spin relaxation time. How much signal is lost depends on the echo time (TE), describing the time at which we sample the current induced in our coil.
We further describe a term T2*, which combines the effects of T2 with local field inhomogeneities and is important when considering gradient echo rather than spin echo imaging. Often, we use the term relaxivity (R1, R2) or inverse of T1 or T2 (R1 = 1/T1, R2 = 1/T2) out of convenience when discussing contrast mechanisms. Of note, both R1 and R2 are magnetic field-dependent properties. At 1.5 T, the T1 of blood is 1200 ms, with a T2 of 150 to 200 ms; the T1 and T2 for fat are 300 and 60 ms, respectively (1).
Contrast Media—Relaxation Enhancement
When a contrast agent is administered, the physics of magnetic resonance described earlier do not change. However, the presence of the contrast agent decreases the observed tissue relaxation times T1 and T2, thereby changing the tissue signal intensity. This also can be expressed as increasing the tissue relaxivities R1 and R2 (i.e., observed 1/T1 and 1/T2), and hence, the effect is termed relaxation enhancement. Because the concentration of protons (water) is much greater (55,000 M) than the concentration of contrast agent (perhaps 0.1 to 5.0 mM), individual contrast complexes must exert relaxation effects on extremely large numbers of water molecules. The efficiency of this process can be expressed as the relaxivity of the contrast agent (Rcontrast), which is linearly related to the concentration of the contrast agent and can be written (11):


where c is the concentration of contrast agent. In other words, the observed relaxivities R1,2 can be thought of as the sum of an intrinsic relaxivity (i.e., the true tissue T1 and T2) and a contrast specific relaxivity proportional to the concentration of contrast present. Contrast agents themselves can be classified as paramagnetic or superparamagnetic, as will be described shortly. As stated, both R1 and R2 depend on magnetic field strength. This effect is demonstrated for five different MR contrast agents in Figure 5-1—a nuclear magnetic relaxation dispersion (NMRD) curve, which in this case plots R1 versus field strength.
Contrast Media—Paramagnetic
Examples of paramagnetic agents include molecular oxygen, free radicals, and the metal ions gadolinium (Gd3+), iron (Fe2+), and manganese (Mn2+). All of these agents are characterized by having relatively large numbers of unpaired electrons. These unpaired electrons generate a locally fluctuating magnetic field, which in turn causes an alteration in tissue T1 and T2.
These complex interactions are often broken into two components: inner sphere and outer sphere. Inner sphere refers to relaxation enhancement resulting from rapid water exchange of the typically single water molecule intimately associated with the contrast complex, and outer sphere refers to the surrounding water solvating the contrast complex (Fig. 5-2) (12).
Of the two, inner-sphere relaxivity (R1,2IS) is typically the larger term, increasing with the square of the contrast agent magnetic moment μeff, which is in turn proportional to the number of unpaired electrons. Thus, ions such as Gd3+, Fe2+, and Mn2+ (with 7, 5, and 5 unpaired electrons, respectively) make better relaxation agents compared with an ion such as Cu2+, the latter with only 1 unpaired electron. Furthermore, inner-sphere relaxivity falls off as the sixth power of the radius between proton and paramagnetic ion (1/r6), making it important to formulate contrast agents such that water can gain close proximity to the paramagnetic center.
A third component to inner-sphere relaxivity relates the quantum mechanical probability of contact interaction between the two, which can be described in terms of rotation, electron spin relaxation, and chemical exchange correlation times, respectively (11,13,14).
Outer-sphere relaxivity (R1,2OS) is basically the same as inner sphere, only the correlation times are altered due to the different properties of outer-sphere water; there is a much larger distance between water and the paramagnetic center (1/r6 dependence); and there exist a vastly greater number of water molecules in that sphere. Nonetheless, the contribution of R1OS can be significant, contributing approximately half the relaxivity for extracellular contrast agents used in medical imaging (12).
Because many metal ions such as Gd3+ alone are highly toxic they must be tightly bound to chelates such as DTPA, DOTA, DTPA-BMA, HPDO3A etc. Manganese (Mn2+) is not as toxic, and there is one FDA approved Mn2+ compound (Mn-DPDP), from which the Mn2+ dissociates in vivo and is taken up by the pancreatic cells and hepatocytes. This compound is typically used for liver and pancreatic imaging.
The prototype gadolinium-based contrast agents, of which there are several (Table 5-1), are termed extracellular fluid agents or ECF agents, as they quickly equilibrate with the extracellular space and do not associate with proteins. These are the agents typically used for MR angiography. Gadopentetate dimeglumine (Gd-DTPA) was the first agent approved for clinical use, and that initial use was for the evaluation of the CNS, particularly tumors. Clinical trials for Gd-DTPA began in 1983, and the agent was approved for human use in 1988 (3).
All of the traditional ECF agents are quite small (<1,000 Da), and all contain one gadolinium ion, an 8-coordinate ligand binding to Gd3+, and a single water molecule coordination site to the gadolinium (the inner-sphere water). Molecular structures demonstrating these properties are shown for two example ECF compounds in Figure 5-2.
Tight binding of Gd3+ to ligands such as DTPA “cages” the gadolinium ion, preventing toxicity, but also reduces the relaxation effect by distancing water molecules from the paramagnetic center (recall the 1/r6 relationship). The coordinated water molecule, however, undergoes rapid chemical exchange (106 exchanges per second) with the solvating outer-sphere water molecules, in effect catalyzing the relaxation process.
The traditional gadolinium ECF agents all have similar relaxivities (Table 5-1), with the R1 and R2 of gadoterate being 3.6 L mmol-1 sec-1 and 4.8 L mmol-1 sec-1, respectively (this and all Table 5-1 relaxivities are measured at 0.5 T—this relates to an artifact of the instrumentation used to measure relaxivities, which only measure up to 20 Mhz, or approximately 0.5 T).
As an example, if gadoterate (0.5 M) were diluted to 1% in the blood (i.e., 5 mmol), we can rewrite Equation [1] for blood as:

where (1/1,200 ms-1) is the R1 of nonenhanced blood (i.e., T1 nonenhanced blood = 1,200 ms). Substituting values into [3], 1/T1 would be 1/1,200 + (3.6)(5) = 18 sec-1. This equates to a T1 of 1/18 seconds or 56 milliseconds, a substantial relaxation effect.
Paramagnetic agents are considered to have a relatively balanced effect between R1 and R2, with all medically used contrast agents having R2 greater than R1. Compare this with superparamagnetic relaxation agents, which are less balanced, having a much greater R2 effect and greater R2/R1 ratio (see below). This makes paramagnetic agents ideal for visualizing blood through shortening T1—that is, the lumen becomes bright on T1-weighted imaging. T2 shortening, on the other hand, results in decreased signal intensity.
Another consideration when discussing the relaxivity of paramagnetic agents is the size of the entire gadolinium complex. Water protons move through regions of paramagnetic influence at a very optimal rate for MRA—slowly enough to experience the relaxation effect, yet fast enough to allow other protons the opportunity to relax as well and thereby magnify the effect (15). If, however, a small gadolinium chelate is bound to a large macromolecule (e.g., MW 50,000 to 250,000 Da), the rotation of the entire complex slows down, increasing the probability of contact between the gadolinium complex and the water proton, and thereby further increasing relaxation enhancement. Examples include gadobenate (Gd-BOPTA), which weakly binds the large protein serum albumin (92,000 Da), leading to an approximate doubling of relaxivity at 0.5 T (16). Another example is gadofosveset (MS-325), which tightly binds
serum albumin (91% bound fraction), resulting in an approximate eightfold increase in relaxivity (17).
serum albumin (91% bound fraction), resulting in an approximate eightfold increase in relaxivity (17).
Contrast Media—Superparamagnetic
Superparamagnetic agents develop large magnetic moments when placed into an external magnetic field—much greater than those seen with paramagnetic agents such as gadolinium (18). Once removed from the magnetic field, they retain no net magnetization. In tissues, superparamagnetic agents create strong local magnetic field inhomogeneities. When protons (water) diffuse through these local regions of field inhomogeneity, this induces phase cancellation (phase incoherence) of spins, decreasing the T2 relaxation time (see Magnetic Resonance Basics section, above) and hence, the signal intensity is decreased (15,18). T1 also decreases, but not as dramatically as does T2. Superparamagnetic agents used for MR are iron oxide based, consisting of a biodegradable coating surrounding the iron oxide core.
Two types of compounds are discriminated based on their aggregate size: large (>50 nm) particles, referred to as a SPIO (superparamagnetic iron oxide) and small (<50 nm) particles, referred to as a USPIO (ultrasmall superparamagnetic iron oxide). Examples of SPIOs include AMI-25 and SHU-555A; USPIOs include AMI-227 and NC100150 (Table 5-1).
Relaxivity of superparamagnetic agents is a function of particle size, with larger particles (SPIOs) having greater relaxivity. More important to consider for vascular MR indications, however, is the ratio of R2 to R1, which tends to be very high for SPIOs (AMI-25: R2/R1 = 160/40 = 4) (18). This means that T2 effects typically dominate T1 effects, and the agents are not appropriate for conventional “bright blood” T1-shortening CE-MRA. Instead, they are used in circumstances where the T2 effect manifesting as signal loss is sought, such as for determining benign from malignant liver lesions and lymph nodes (19,20,21). USPIOs such as AMI-227 have much more favorable R2/R1 ratios (R2/R1 = 53/23 = 2.3), making them more appropriate as T1-shortening agents but still not as ideal as the paramagnetic agents (22,23).
Contrast Pharmacokinetics and Biologic Factors
In order to properly use MR contrast agents, it is important to understand their formulation, biodistribution, and elimination pathways. Injectable MR contrast agents for MRA use must be formulated at adequate concentration to effectively alter relaxation at achievable blood concentrations and must be either soluble or homogeneously dispersible in blood. Typically, 0.1- to 1.0-M formulations are employed, depending on agent relaxivity (Table 5-1).
Another consideration is viscosity, which must be low enough that bolus injection can be performed through standard-size intravenous (IV) catheters (acceptable range is approximately 0 to 3 centipoise) (11). Warming the contrast agent to body temperature reduces viscosity, which can be useful when injecting high-viscosity agents such as gadopentetate dimeglumine through smaller IVs (24,25).
Osmolality must also be considered, as injection of a sufficient quantity of a hyperosmolar agent can transiently increase serum osmolality (normal serum osmolality is approximately 290 mOsm/kg), which in turn leads to diffusion of extracellular water into the intravascular space. Under extreme circumstances, passage of a high-osmolality contrast bolus can, in theory, disrupt the blood–brain barrier with serious consequences (26). Acceptable contrast agent osmolalities range from 0.3 to 2.0 Osm/kg (11).
The relatively small quantities of MR contrast used (20 to 40 cc) make the issue somewhat less important than is the case of contrast CT, where boluses of 150 to 200 cc are common. The small volumes of MR contrast translate into a low osmotic load (volume × the osmolality) delivered to the patient, as will be discussed later.
Additionally, injectable compounds must be stable in vivo (or be metabolized to nontoxic products), the compound (as well as any additional MRI-inactive materials added) must be of acceptable toxicity and completely eliminated from the body in a reasonable amount of time, and the compound must have a usefully long shelf life. Finally, when considering gadolinium agents, the toxic gadolinium ion must remain tightly bound to the ligand (see Magnetic Resonance Contrast Safety, below).
The biodistribution of a contrast agent is governed by the kinetics of distribution, clearance, and excretion. Most of the data on MR contrast biodistribution comes from the gadolinium ECF contrast agents gadopentetate (3,27) and gadoterate (28), although the other ECF agents are considered to behave in a relatively similar fashion. In terms of kinetics and imaging behavior, the ECF compounds (Table 5-1) are considered interchangeable, with only slight variations in physical parameters among them (1,12).
The two-compartment model provides the basis for understanding and discussing MR contrast kinetics. In this model, the intravascular space (arteries and veins) makes up one compartment, while the extracellular extravascular space (EES) makes up the other. Contrast agents have varying abilities to diffuse back and forth between the two compartments (ECF agents diffuse rapidly; blood pool agents and SPIOs diffuse slowly). Initially, most of the flux is from the intravascular space into the EES due to the high intravascular concentration after bolus injection. For ECF agents, equilibrium between the two compartments occurs
quite rapidly (in a matter of minutes), providing one reason why CE-MRA is optimally performed during the “first pass” of contrast. A generalized kinetic model of contrast dynamics can be written (29):
quite rapidly (in a matter of minutes), providing one reason why CE-MRA is optimally performed during the “first pass” of contrast. A generalized kinetic model of contrast dynamics can be written (29):

where Ct is the EES concentration, Cp is the plasma (intravascular) contrast concentration, Ktrans is the volume transfer constant describing contrast transfer between the plasma and EES, and κep is the rate constant describing contrast transfer between the EES and plasma. A simple diagram demonstrating this model is shown in Figure 5-3. This is simply a mathematical way of saying that the rate by which concentration changes in the EES (dCt/dt) increases proportional to the intravascular contrast concentration (Cp), while at the same time decreases at a rate proportional to the EES contrast concentration (Ct). Of course, Cp also decreases according to how the contrast is eliminated (e.g., renal or hepatic clearance).
Another way to describe contrast kinetics uses a biexponential model of elimination half-lives. This takes into account the two-compartment model in combination with clearance/excretion and is most influenced by physical contrast agent size and the differences in capillary endothelium between different organs. In muscle and connective tissue, the endothelium forms a continuous layer around the capillary, preventing all but the smallest molecules (such as ECF agents) from leaking out. In the kidney, however, the glomerular capillaries have a fenestrated enodothelium with pores measuring 60 to 70 nm in diameter. This allows molecules less than 20,000 Da in weight to pass freely and molecules up to 70,000 Da to pass depending on lipid solubility, polarity, and pH (22).
In this model, two half-lives are defined; the distribution half-life (T1/2α), describing the rate by which contrast agent distributes out of the intravascular space, and the elimination half-life (T1/2β), describing the rate of elimination from the body. These concepts are diagrammed in Figure 5-3. ECF agents are known to rapidly distribute throughout the EES via capillaries (excepting neural tissue due to the blood–brain barrier), as they are all small molecules less than 1,000 Da in size. In fact, it is estimated that 50% of gadopentetate passes from the intravascular space to the EES on the initial pass through the capillaries (30). The half-life values for the ECF agents are well described and relatively similar, with T1/2α ranging from 3.6 to 12 minutes, and T1/2β ranging from 1.3 to 1.6 hours (1,28).
In comparison, blood pool agents such as gadofosveset and B22956, which reversibly bind serum albumin, have much longer elimination half-lives (e.g., gadofosveset T1/2β estimated at 2 to 3 hours) since the large complex that is formed cannot be filtered through the glomerulus (31). Distribution half-life, however, is very similar to that of the ECF agents (T1/2α 3 minutes for gadofosveset (22)), as the unbound fraction is free to pass through the capillary endothelium.
The macromolecular agents such as Gadomer-17 and P792 have somewhat different pharmacokinetic properties, with a long distribution half-life but a relatively rapid elimination half-life (due to swift glomerular filtration). This means that nearly all the contrast present remains intravascular, but it is rapidly cleared from the bloodstream.
The iron oxides have different properties yet, with the larger SPIOs having quite rapid elimination half-lives (8 to 10 minutes) due to rapid sequestration by liver Kupfer cells (18) and USPIOs having much longer elimination half-lives (90 minutes in the case of NC100150) (32,33).
A third parameter, the steady state distribution volume (Vd ss) can also be calculated using this model, describing the total intra- and extravascular volume over which a contrast agent distributes. Typical distribution volumes range from 171 mL/kg for gadopentetate to 256 mL/kg for gadoterate (28).
Consider again the example in Equation [3], where gadoterate (0.5M) is injected to achieve a 1% arterial phase blood concentration, resulting in a peak T1 of 56 milliseconds. If the total injected volume is 20 cc (into a 75-kg person), and the distribution volume is approximated as 200 mL/kg, the equilibrium concentration (discounting elimination) would be (20 cc × 500 mmol/L)/(200 mL/kg × 75 kg) = 0.66 mmol. Putting this value into Equation [3], the equilibrium T1 (EES and intravascular space) is approximately 420 milliseconds, which is much greater than that of fat (270 ms); this example demonstrates that in order for the T1 of blood to be shorter than the T1 background tissue, imaging must be performed in the first-pass arterial phase.
Elimination pathways vary according to the type of contrast agent. The gadolinium ECF agents, small and extremely hydrophilic, are predominately eliminated unmetabolized via a renal route—glomerular filtration, active secretion, or a combination of the two. As stated earlier, elimination half-lives are on the order of 90 minutes. The only exception to pure renal elimination is gadobenate (Gd-BOPTA), which is partially taken up by the hepatocytes and excreted in bile (34). This makes it useful for hepatic imaging in addition to MRA (35).
Gadolinium-based blood pool agents, on the other hand, remain intravascular for a much longer time and are eliminated from the body much more slowly (longer T1/2β). These agents remain largely intravascular due to one of two mechanisms: (a) a substantial fraction (>80% at any one time [36]) reversibly binds to serum albumin, or (b) the large inherent size (>20,000 Da) of the blood pool agent prevents it from diffusing through the endothelium.
In the first case, because albumin is not eliminated by the healthy kidney, it is only the small unbound (free) fraction of contrast agent that is available for renal excretion (17). In the second case, the macromolecule is large enough to remain intravascular but small enough to be excreted by glomerular filtration (37).
Iron oxide agents (i.e., SPIOs, USPIOs) are metabolized in a completely different fashion from the gadolinium agents. These agents are cleared by the body’s reticuloendothelial system (RES), which includes the liver, spleen, bone marrow, and lymph nodes, with the Kupfer cells within the liver being responsible for approximately 80% of the clearance (18). How fast this RES clearance occurs depends on blood half-life, which is in turn dependent on particle size. The larger SPIOs are cleared rapidly from the blood (T1/2β = 8 to 10 minutes), while the smaller USPIOs are not immediately recognized by the RES and have longer elimination half-lives (T1/2β = 45 to 200 minutes) (18,32).
While all of the iron oxide aggregates are large enough that they remain predominantly within the intravascular space, only the USPIOs are considered candidates for blood pool MRA. This is not only due to the longer blood half-life of USPIOs, but is also due to their more favorable R2/R1 ratio (see Contrast Media—Superparamagnetic, discussed earlier). The SPIOs appear better suited to hepatic and lymph node imaging.
Magnetic Resonance Contrast Agent Safety
Medical imaging contrast agents are considered pharmaceuticals and are therefore regulated by the FDA. As with therapeutic drugs, new imaging contrast agents need to be proven efficacious and safe through a specific and structured process that is overseen by the FDA prior to being approved for human use. In this process, the initial assessment of safety is performed using in vitro and animal studies to establish the toxicity and dose tolerance of the agent (38).
A frequently stated measure of preclinical safety that is established in these tests is the median lethal dose (LD50), which describes the dose of a drug that results in the acute death of approximately half the animals in the study population (39). Though such measures as the LD50 have been correlated with the incidence of idiosyncratic reactions with iodinated contrast media, these types of animal and in vitro measures of toxicity typically do not have well-defined clinical value owing to the significantly lower doses used clinically. These toxicity measures are useful, however, in that they provide a means to compare the theoretical tolerances of different agents (40).
Once the initial safety is established, subsequent studies are performed on humans (Phase I–III trials) to further define the efficacy and tolerance of the agent. On completion of Phase III, the product label is developed, which summarizes the experience with the drug and provides specific guidelines for its use, and the agent is submitted to the FDA for approval. If approved, the drug can then be marketed for its designed application, at which time it enters Phase IV, or the postmarketing stage of development (38).
Currently, there are five widely available FDA-approved gadolinium-based MR contrast agents available in the United States (gadopentetate dimeglumine, gadoteridol, gadodiamide, gadoversetamide, and gadobenate dimeglumine)—the first four representing the conventional extracellular fluid (ECF) gadolinium chelates (41). Though the
information describing the safety of these agents obtained from the trials and studies performed during the approval process demonstrate that these agents are exceptionally well tolerated and do not exhibit nephrotoxicity at approved doses, life-threatening reactions can still occur (42,43,44,45).
information describing the safety of these agents obtained from the trials and studies performed during the approval process demonstrate that these agents are exceptionally well tolerated and do not exhibit nephrotoxicity at approved doses, life-threatening reactions can still occur (42,43,44,45).
Thus, the MR physician prescribing these pharmaceutical agents must be (a) aware of the potential adverse effects, (b) able to identify patients at increased risk for contrast reactions, and (c) capable of managing a contrast reaction (41,46). As previous sections of this text have presented principles regarding the effectiveness of contrast agents related to their relaxivity and biologic distribution, this section will discuss issues related to contrast agent toxicity and contrast use in special patient populations. Safety-related knowledge should assist the practitioner in understanding subtle differences between the various agents, enabling selection of the most efficacious and safe compounds from a continually growing array of clinically available agents and formulations (46).
Etiology of Contrast Media Reactions
Adverse reactions to contrast media can be classified as anaphylactoid, nonanaphylactoid, or a combination of the two. Anaphylactoid (idiosyncratic) reactions occur unexpectedly, vary in severity, and symptomatically appear similar to anaphylactic reactions. While their precise mechanism is unknown, it is believed to be related to the release of vasoactive substances that subsequently result in systemic vasodilation and/or bronchospasm. The nonanaphylactoid reactions include those that are related to chemotoxic, osmotoxic, and vasovagal mechanisms and do not have the features of an anaphylactoid response. Chemotoxicity refers to the inherent toxicity related to the chemical structure of the compound. Osmotoxicity reflects osmotic effects and the ability to induce water movement across semipermeable membranes. Vasovagal reactions are initiated in the central nervous system and reflect increased vagal tone on the heart and blood vessels (47,48).
Studies into the mechanisms of adverse reactions of iodinated contrast agents hypothesize that the primary pathogenic processes involve cell membrane destabilization and the subsequent release of vasoactive substances or activation of the complement system. What is known from experience with the iodinated contrast media is that the use of low-osmolar, nonionic agents results in nearly a fivefold decrease in the incidence of adverse reactions when compared with ionic agents (47).
Pharmacodynamically, it is postulated that this lower reaction rate with the nonionic compounds is related to the greater number of hydroxyl groups that these agents contain. The hydroxyl groups attract water, thereby forming a shielding shell of bound water that decreases interactions with hydrophobic segments of proteins and cell membranes (48). Other described nonanaphylactic reactions of iodinated contrast media include chemotoxic renal tubular injury, osmotoxic-induced changes in blood flow and vascular permeability, vasodilation, and the pain that occurs when hyperosmolar agents are administered during arteriography (48,49).
Safety of the Extracellular Fluid Gadolinium Chelates
In comparison to the iodinated contrast media, the gadolinium-based MR agents possess some unique safety issues. The principal chemotoxic concern with gadolinium is that the free ion is extremely toxic (50), with an LD50 in rodents between 0.3 and 0.5 mmol/kg (22). When administered intravenously, the free ion is distributed primarily to the liver, where it forms insoluble colloid particles. Elimination of gadolinium is also extremely slow, with a biologic half-life of several weeks (51).
The unfavorable pharmacologic parameters of the free gadolinium ion are significantly improved by noncovalently linking the gadolinium ion to a chelating molecule such as DTPA, which modifies the biodistribution, elimination, and toxicity of the resulting ion-ligand complex (50). Interestingly, while the four conventional gadolinium chelates approved for use in the United States (gadopentetate dimeglumine, gadoteridol, gadodiamide, and gadoversetamide) differ in the structure of the chelating molecule, they have remarkably similar pharmacokinetics, effectiveness, and safety profiles (41).
These four agents are considered equally efficient as extracellular contrast agents, with no significant difference between their T1 and T2 relaxivities (Table 5-1). They are all labeled as interstitial agents with an apparent volume of distribution (Vd ss) equivalent to the extracellular fluid compartment (200 to 300 mL/kg) (22). Excretion for each compound is primarily by the kidneys, with biologic elimination times being approximately 1.5 hours (51).
Though very similar pharmacokinetically, there are several pharmacologic differences between the four conventional FDA-approved agents that are theoretically important with regards to safety. Given the extreme toxicity of the free gadolinium ion, the agents that are more stable in vivo, and therefore better able to maintain the gadolinium ion-chelating ligand complex, are considered to have a safety advantage (41).
In general, the macrocyclic ring chelate (gadoteridol—see Fig. 5-2) is more stable than the linear agents (gadopentetate, gadodiamide, gadoversetamide), as demonstrated by
their thermodynamic stability constants—an in vitro measure of the affinity between the metal ion and the ligand (50) (Table 5-2). The higher stability also reduces the risk of transmetalation, in which other metal ions (e.g., copper and zinc) compete for gadolinium attachment sites, allowing the release of free gadolinium ions. Despite these hypothetical risks, no harmful affects in humans have been reported due to free gadolinium ion deposition resulting from clinical use (41).
their thermodynamic stability constants—an in vitro measure of the affinity between the metal ion and the ligand (50) (Table 5-2). The higher stability also reduces the risk of transmetalation, in which other metal ions (e.g., copper and zinc) compete for gadolinium attachment sites, allowing the release of free gadolinium ions. Despite these hypothetical risks, no harmful affects in humans have been reported due to free gadolinium ion deposition resulting from clinical use (41).
The ECF gadolinium chelates also differ in their osmolality and viscosity (Table 5-2). Gadoteridol and gadodiamide have the lowest osmolality and viscosity, in part since they are nonionic. Gadopentetate dimeglumine has the highest osmolality and viscosity. Gadoversetamide is intermediate between the other two groups (41). When comparing these agents with iodinated contrast media, it is important to recognize that the manifestation of osmotoxicity is dependent on the osmotic load that the patient is exposed to rather than the measured in vitro osmolality. This relates to the drug osmolality, volume of drug administered, and the volume of distribution.
For example, the total osmotic load for an injected volume of 14 cc of Gd-DTPA (1960 mOsmol/kg) is 27 mOsmol, which compares with an osmotic load of 104 mOsmol for a 150-cc dose of iohexol (690 mOsmol/kg) (52). Thus, while low osmolality is an important safety parameter for iodinated contrast agents, it is less of a factor with these gadolinium chelates, given the lower volumes and injection rates traditionally employed for MR studies (41). Table 5-3 compares the osmotic loads that result from the administration of some commonly used MR and CT contrast media.
Potential Adverse Events
Multiple variables and numerous differences in study design—such as the phase of development, number of participating sites and their locations, study indications, and selected end points—make it extremely difficult to perform direct comparisons of the overall incidence of adverse reactions derived from various clinical trials performed for each agent (46). For example, the reported incidence of adverse events for gadopentetate in the central nervous system ranges between 6.0 and 19.9%, while it has been reported as high as 44.1% when applied to liver pathology. This is confounded when one includes the finding that the reaction rate with a saline placebo in a different liver study was 44.2% (46).
General comparisons between agents, however, can be made through the use of parallel-group or patient crossover studies that compare agents directly with one another in the same study (53). What these comparative studies have shown is that there are no discernable differences in the incidence of adverse events between the available ECF gadolinium chelates. As a result, most references to safety are based on the data obtained with gadopentetate dimeglumine, the first agent approved for use in the United States and the one that has been the most extensively studied (53).
Available estimates place the overall incidence rate of adverse events for ECF gadolinium agents at less than 5%, with the incidence of any single adverse event occurring being 1% or less (51). The most frequent reactions that occur with these agents are nausea with or without emesis (25% to 42% of reactions), headache (18%), urticaria (3% to 7%), and taste perversion (<1%) (46,54). Similar to the iodinated agents, numerous other associated reactions from hot flashes to gingivitis have been reported involving every organ system, though at frequencies much less than 1% (51).
Despite that the vast majority of adverse reactions to these MR contrast agents are mild, and reactions requiring any form of treatment are rare (54), severe anaphylactoid reactions can and do occur (41). Reported incidences of severe reactions range from 1 in 10,000 to 1 in 500,000 doses (41,51), which is still low even when compared with the rates quoted for low osmolar iodinated CT contrast media (1.6 in 10,000) (54). The potential for life-threatening reactions necessitates that MR personnel be trained to handle adverse events, and that resuscitation equipment be available whenever these medical imaging agents are administered.
While the exact mechanisms behind these complications are not clearly understood, the similar characteristics of adverse events that occur with both MR and iodinated contrast media has been used to support the use of the empiric therapy employed with iodinated contrast media for treating MR contrast reactions (52,54). Specific treatment protocols are available from the Committee on Drugs and Contrast Media of the American College of Radiology (54).
Safety Considerations with Special Patient Populations
As with the administration of iodinated contrast media, the safe administration of a gadolinium-based MR agent may need to be modified or require preprocedural attention in selected patient populations. These special groups include (a) patients with renal insufficiency/failure, (b) persons who present an increased risk for a reaction due to atopy or prior reaction history, (c) people with sickle cell disease or a hemolytic anemia, (d) patients who are pregnant, (e) women who are breast-feeding, (f) children less than two years of age, and (g) those at risk for contrast extravasation.
Renal Insufficiency/Failure
Patients with decreased renal clearance stand a greater risk of dissociation and toxicity due to the longer half-life and
subsequent longer in vivo exposure to contrast agents. This is related to the concerns regarding nephrogenic systemic fibrosis (NSF), and that entity is discussed more in depth in Chapter 22. With normal renal function, the rapid excretion and high stability of the gadolinium chelates present almost no risk of dissociation. While all four conventional ECF gadolinium chelates are cleared entirely by urinary excretion, none have been found to exhibit nephrotoxicity at approved doses in patients with impaired renal function, when administered intravenously (41,55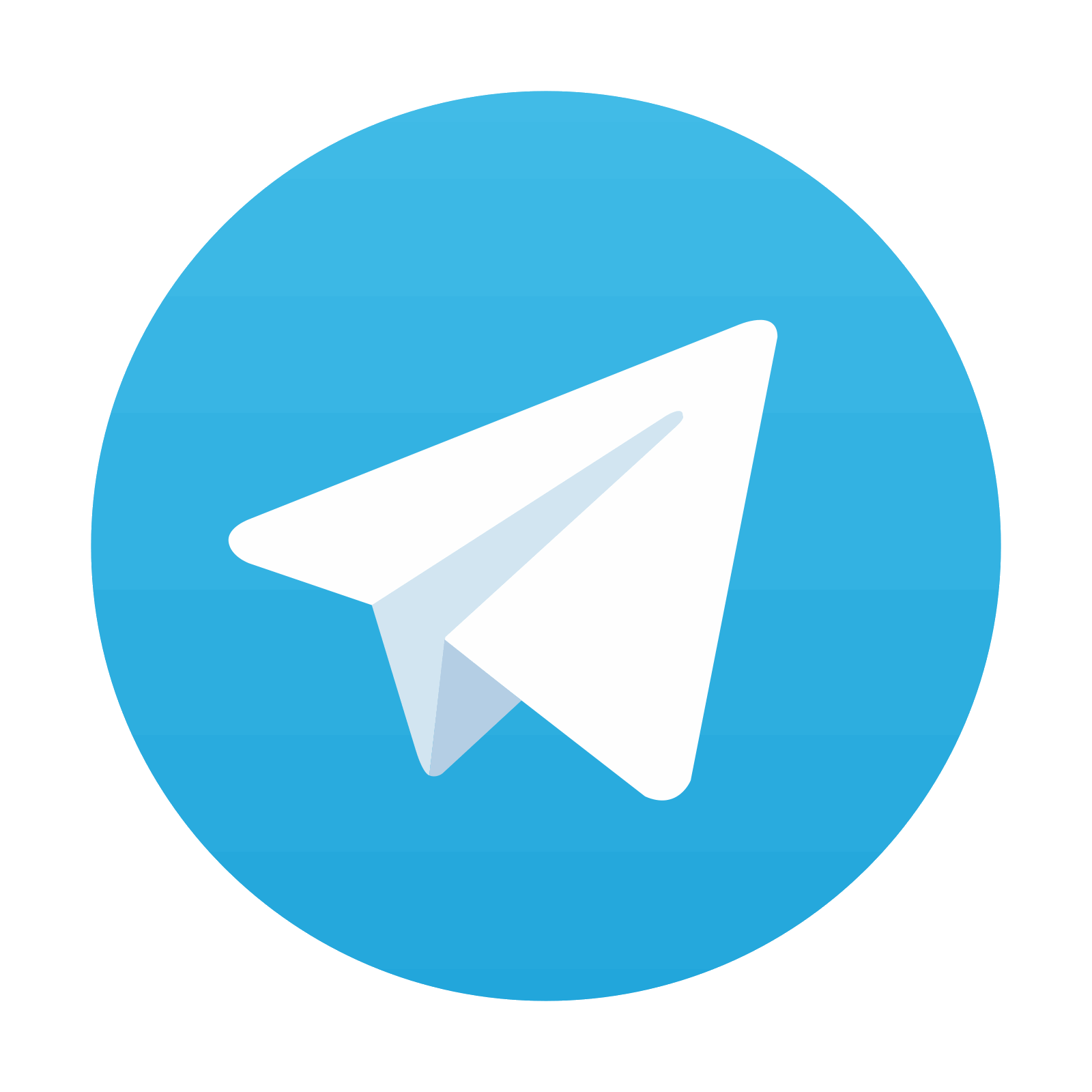
subsequent longer in vivo exposure to contrast agents. This is related to the concerns regarding nephrogenic systemic fibrosis (NSF), and that entity is discussed more in depth in Chapter 22. With normal renal function, the rapid excretion and high stability of the gadolinium chelates present almost no risk of dissociation. While all four conventional ECF gadolinium chelates are cleared entirely by urinary excretion, none have been found to exhibit nephrotoxicity at approved doses in patients with impaired renal function, when administered intravenously (41,55
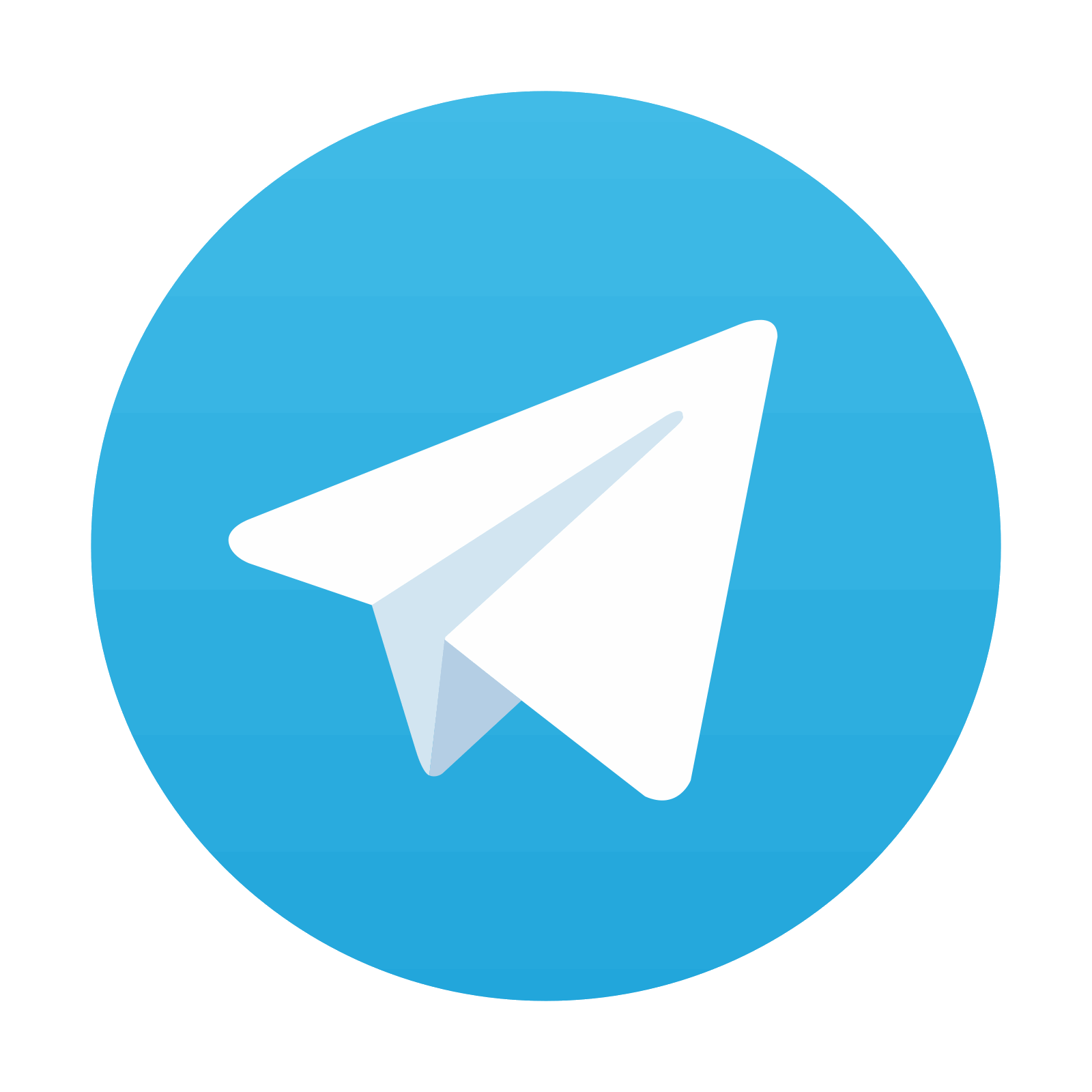
Stay updated, free articles. Join our Telegram channel
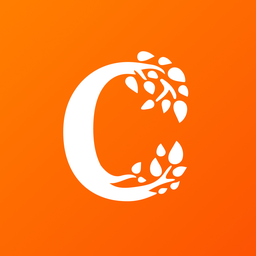
Full access? Get Clinical Tree
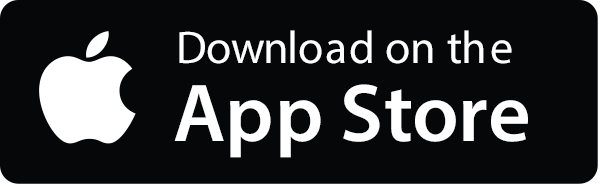
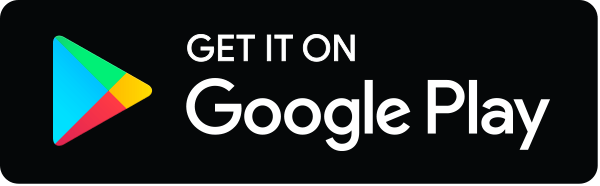
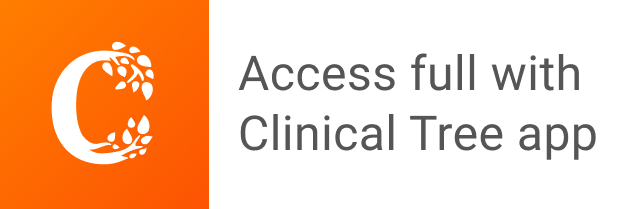