Atherosclerosis: In Vivo Characterization
Alexander W. Leber
Venkatesh Mani
Juan Gilberto S. Aguinaldo
Zahi A. Fayad
Atherosclerosis is a chronic inflammatory disease that affects essentially all arterial beds of the human vascular system. It is the main cause of death in the Western Hemisphere, due to cardiovascular syndromes such as myocardial infarction (MI), heart failure, and cerebrovascular accidents. It derives from a complex interaction between genetic and environmental factors with inflammatory stimuli altering the structure of the arterial wall. An improved understanding of the disease process along with the emerging capability to noninvasively assess this process may facilitate preventative strategies for atherosclerosis or the identification of patients at risk for acute events.
Coronary Atherosclerosis Imaging
Despite advances in our understanding of the pathogenesis of atherosclerosis, coronary heart disease is still the leading cause of death in Western societies. Approximately 50% of all MIs occur in patients with no prior symptoms. It is well established that the risk for plaque rupture is predicated by plaque burden and plaque composition (1). Reliable and accurate assessment of the composition of coronary atherosclerosis currently can be achieved mainly by invasive methods like intracoronary ultrasound or angioscopy (2). Since
these are invasive procedures, they are not suitable for preventive investigations in asymptomatic patients.
these are invasive procedures, they are not suitable for preventive investigations in asymptomatic patients.
Electron beam computed tomography (EBCT) and multidetector computed tomography (MDCT) enable an accurate noninvasive identification and quantification of calcified coronary plaques (3). Although calcified plaques reflect only a small proportion of the entire plaque burden and the proportion of lesions containing calcium reveals a broad variability among humans, it is suggested that the extent of coronary calcium is a surrogate marker for total plaque burden. It has been demonstrated that future coronary events may be predicted on the basis of the calcium score derived by various CT modalities (4,5).
However, MI is initiated by rupture or superficial erosion of vulnerable coronary plaques, and these plaques are not necessarily calcified, as calcium is considered to be a frequent feature of stable lesions (1). Further, there are several potential morphologic features such as lipid cores that constitute potential targets for noninvasive imaging in particular for multislice CT and magnetic resonance imaging (MRI). Hence, for risk stratification and guidance of antiatherosclerotic therapies, a noninvasive tool that can identify both calcified and noncalcified plaques would be of great interest.
Clinical Value of Coronary Atherosclerosis Imaging
Coronary atherosclerosis starts very early in life, and early stages of atherosclerotic lesions are already present in young adults under 20 years of age. In general, the disease remains clinically silent for years—50% of acute MIs occur in previously asymptomatic patients as the first clinical manifestation of coronary artery disease (CAD).
In other patients, the first clinical sign of CAD is typically stable angina pectoris, which is due to myocardial ischemia from a high-grade coronary lumen obstruction. The reason for the late development of clinical symptoms is that plaque accumulation within the vessel wall leads to compensatory diameter expansion, a process called coronary vessel remodeling (6). Therefore diagnostic tests targeting the detection of myocardial ischemia due to high-grade coronary stenosis identify only the late development stage of coronary atherosclerosis.
Currently, catheter-based invasive modalities like intravascular ultrasound (IVUS) and angioscopy are almost exclusively used for identification of coronary atherosclerosis in a preclinical stage. IVUS has been shown to allow an accurate determination of coronary plaque burden and plaque composition (compared with histology).
From postmortem histopathologic studies investigating the coronaries of victims of MI, it is known that the responsible culprit lesion in most cases reveals typical characteristics: It is an eccentric plaque containing a large lipid core that is covered by a very thin fibrous cap with an abundance of inflammatory cells (macrophages) on its shoulders. Calcium is not necessarily present (7).
In accordance to these histologic criteria, IVUS has shown that plaque rupture initiating MI occurred most frequently in plaques with a large plaque volume with hypo-echoic tissue revealing echolucent zones and presenting extensive compensatory vessel remodeling (8). Interestingly, most of these plaques caused no significant luminal obstruction on initial angiograms.
Evidence from recent investigations suggests that plaque rupture is a systemic coronary process rather than a focal event. In a multivessel IVUS study of patients with MI, Riofoul et al. (9) identified multiple silent plaque ruptures in vessels distinct from the culprit lesion. Similar observations were made by angioscopy, where multiple yellow (lipid-rich) lesions were found in patients with acute coronary syndromes, whereas in patients with stable coronary artery disease, the predominant plaque type was a white (fibrotic, calcified) plaque (10).
Goldstein et al. reported the presence of multiple complex lesions in patients with acute MI (11). In accordance, Leber et al. found significantly more noncalcified and less calcified lesions by multislice CT in patients with acute MI as compared with patients with stable coronary artery disease (12). Those studies indicate that it may not be sufficient to identify the one vulnerable plaque that will cause MI in the future because patients at risk have several of them. Moreover, it is necessary to identify the vulnerable patient on the basis of the “pan-coronary” morphology of atherosclerosis.
The reasons for the sudden rupture of multiple plaques are not fully understood at the present time. It is suggested that systemic inflammatory factors play a key role in the development of vulnerable plaques and their progression to rupture. Therefore, a combined approach of determining plaque burden and plaque composition in conjunction with the determination of biomarkers like CRP or s-CD40 ligand will play an important role for risk stratification in the future.
Computed Tomographic Imaging of Coronary Plaque—Technical Aspects
The rapid motion of the beating heart presents a challenge to acquiring high-quality motion and artifact free images. In the past, CT failed to generate diagnostic-quality images because of its restricted temporal resolution. Former spiral CT technology needed at least 500 ms to obtain one tomographic slice. With the introduction of electron beam CT (EBCT) and its fast acquisition time of 100 ms, the first motionfree images of the coronaries were obtained. Albeit, the excellent temporal resolution motion artifacts can only be avoided in a
certain phase of the heart cycle during diastole. Thus, irrespective of the heart rate, it is mandatory to generate images at identical time points of the R-R interval, which is achieved by using an electrocardiogram (ECG) trigger.
certain phase of the heart cycle during diastole. Thus, irrespective of the heart rate, it is mandatory to generate images at identical time points of the R-R interval, which is achieved by using an electrocardiogram (ECG) trigger.
With the introduction of MDCT, a temporal resolution of 83 to 250 ms is now available. In addition to the faster gantry rotation, the major advantage of this technology compared with conventional mechanical spiral CT scanners is that it consists of 4 to 32 detector rows, which allow generation of 4 to 64 slices simultaneously. For coronary applications, the whole volume of the heart is covered in the spiral technique with simultaneous digital registration of the ECG signal. Using this approach, images can be reconstructed after data acquisition retrospectively at every time point of the ECG cycle. The net result is a robustness against extra systoles and arrhythmias. Furthermore, different trigger points can be tailored to each coronary vessel.
CT scanners using 16- and 64-slice technology offer a very high spatial resolution and generate very thin slices, allowing the acquisition of isotropic voxels. This has already led to a major advance in noninvasive coronary angiography. For visualizing noncalcified plaques (unlike calcium scoring), a contrast agent has to be administered intravenously, and specific protocols for coronary computed tomography angiography (CTA) are applied.
To obtain diagnostic image quality with MDCT, it is essential to reduce heart rates below 65 beats per minute (bpm), generally accomplished by administering oral or intravenous beta-blockers. However, slowing the heart rate sufficiently can be a challenge, highlighted by the results in a recent study where 15% of patients failed to achieve a sufficient reduction in heart rate despite beta-blockade (13). Furthermore, patients with renal insufficiency or those allergic to contrast agent cannot be investigated by MDCT. Therefore, CTA is not suitable for a considerable number of patients.
Table 8.1 Sensitivity of Multidetector Computed Tomography in the Detection of Different Coronary Plaques in Vessels (58/68) and Specificity to Exclude Coronary Lesions | ||||||||||||||||||||||||||||||||||||||||||||||||||||||||||||||||||||||
---|---|---|---|---|---|---|---|---|---|---|---|---|---|---|---|---|---|---|---|---|---|---|---|---|---|---|---|---|---|---|---|---|---|---|---|---|---|---|---|---|---|---|---|---|---|---|---|---|---|---|---|---|---|---|---|---|---|---|---|---|---|---|---|---|---|---|---|---|---|---|
|
Computed Tomographic Imaging of Noncalcified Coronary Plaque
Plaque Composition
The recent advances in CT technology allow for the detection and classification of many atherosclerotic lesions affecting the coronary arteries. The CT attenuation of plaque components correlates well with the echogenicity of ultrasound and even histopathologic criteria. However, only limited data concerning CT imaging specific to the coronary plaque exists.
A recent ex vivo study by Becker et al. showed that advanced stages of coronary plaques in heart specimens can be detected by MDCT (Fig. 8-1) (14). In their comparison with the histologic Stary classification (Fig. 8-1A), they found that CT could visualize type III to type VI plaque, whereas early stages (types I and II) were not detectable.
In a 4-slice CT study, Schroeder et al. found a good correlation between the CT attenuation measured within coronary plaques and the echogenicity of plaques on IVUS (15). In another recent study, Leber et al., using a 16-slice CT, demonstrated that noncalcified lesions could be detected with a reasonable sensitivity of 78% (Table 8-1).
However, they found that the ability of 16-slice CT to identify noncalcified plaques is restricted to larger, more advanced lesions (with a plaque diameter of at least 1.5 mm) that are located in proximal and middle coronary segments, limitations related to temporal resolution (13). Quantitative characteristics of MDCT detected versus nondetected coronary plaques are shown in Table 8-2. Similar results were also reported by Achenbach et al. (16).
However, they found that the ability of 16-slice CT to identify noncalcified plaques is restricted to larger, more advanced lesions (with a plaque diameter of at least 1.5 mm) that are located in proximal and middle coronary segments, limitations related to temporal resolution (13). Quantitative characteristics of MDCT detected versus nondetected coronary plaques are shown in Table 8-2. Similar results were also reported by Achenbach et al. (16).
In accordance with previously reported results, Leber et al. also found a good correlation between CT density
measurements within plaques and echogenicity on IVUS. Hypoechoic plaques on IVUS that represented lipid-rich plaques had a significantly lower density than fibrotic plaques. Corresponding longitudinal and axial IVUS and MDCT images are shown in Figure 8-2. However, as in ex vivo studies, they also observed a wide overlap of density values among fibrous and soft plaques, making the differentiation of these lesions very difficult.
measurements within plaques and echogenicity on IVUS. Hypoechoic plaques on IVUS that represented lipid-rich plaques had a significantly lower density than fibrotic plaques. Corresponding longitudinal and axial IVUS and MDCT images are shown in Figure 8-2. However, as in ex vivo studies, they also observed a wide overlap of density values among fibrous and soft plaques, making the differentiation of these lesions very difficult.
Table 8.2 Quantitative Characteristics of Multidetector Computed Tomography Detected vs. Nondetected Coronary Plaques | |||||||||||||||
---|---|---|---|---|---|---|---|---|---|---|---|---|---|---|---|
|
There are several reasons for this observation: (a) Even by IVUS analysis, the separation between lipid-rich and fibrous tissue is difficult, as the echogenicity differences between these plaques are relatively small. (b) Density values measured within plaques vary depending on the CT-attenuation within the lumen. The optimal luminal contrast enhancement for plaque differentiation has been found to be within 200 and 250 HU. This value however cannot be consistently achieved in clinical practice. (c) Coronary plaques are rarely composed only from fibrous, calcified, or lipid-rich tissue. In the majority of cases, all kinds of tissue can be observed. CT density values for hypoechoic, hyperechoic, and calcified plaques are shown in Figure 8-3.
Vessel Remodeling
As mentioned previously, positive coronary vessel remodeling is supposed to be a characteristic feature of vulnerable plaques. Schoenhagen et al. have demonstrated that MDCT offers the opportunity to visualize this compensatory diameter expansion (17). Achenbach et al. have also demonstrated that in highly selected patients, the 16-slice CT can determine positive and negative vessel remodeling, and it is feasible to accurately determine plaque areas (18).
The limitation of all these quantitative CT analyses, however, is that they were all exclusively performed in patients with high CT-image quality. This high quality
could only be achieved in approximately 75% of patients. Nevertheless, these study results indicate that MDCT provides a unique opportunity to identify several morphologic features associated with plaque vulnerability like plaque composition, plaque volume, and positive vessel remodeling. Although the prognostic impact of these features is unknown so far and the first follow-up studies are just under way, evidence from first clinical studies underline the predictive potential of MDCT.
could only be achieved in approximately 75% of patients. Nevertheless, these study results indicate that MDCT provides a unique opportunity to identify several morphologic features associated with plaque vulnerability like plaque composition, plaque volume, and positive vessel remodeling. Although the prognostic impact of these features is unknown so far and the first follow-up studies are just under way, evidence from first clinical studies underline the predictive potential of MDCT.
In a clinical study, MDCT-derived plaque morphology of patients with acute myocardial infarction (AMI) and stable angina pectoris was compared. In this population, patients with stable angina had significantly more calcified and less noncalcified lesions than patients with MI.
As a consequence, total plaque burden of patients with MI would have been significantly underestimated by calcium scoring alone. Moreover, in 10% of patients with AMI, only noncalcified lesions were present. These observations imply that noncalcified lesions may be involved in the process leading to unstable coronary disease.
As a consequence, total plaque burden of patients with MI would have been significantly underestimated by calcium scoring alone. Moreover, in 10% of patients with AMI, only noncalcified lesions were present. These observations imply that noncalcified lesions may be involved in the process leading to unstable coronary disease.
Schroeder et al. have demonstrated that the prevalence of noncalcified lesions is inhomogeneous even among a patient selection with a similar high-risk profile, which might reflect different prognostic outcomes. They observed in 14% of patients only noncalcified lesions, in 50% calcified and noncalcified lesions, and in 36% of patients only calcified lesions. However, future prospective studies comparing these populations are needed to assess the relative risk for developing adverse coronary events.
Future Developments in Computed Tomographic Imaging
Due to a rapid improvement of the new generation submillimeter multislice CT technology, noninvasive tomographic imaging of the coronary vessel wall has now become a reality. The early clinical studies have shown the ability of 16-slice CT to determine plaque burden, plaque composition, and compensatory vessel wall remodeling. These novel findings already constitute an important step forward in assessing coronary atherosclerosis noninvasively in a detailed manner that opens promising new opportunities for a better understanding and risk stratification of coronary atherosclerosis. Current limitations, mainly the insufficient accuracy to detect small lesions in distal coronary segments, might be overcome by improved spatial and temporal resolution of the next generation of CT scanners operating with 64 and more detectors.
Magnetic Resonance Imaging of Coronary Plaque—Technical Aspects
Compared with CT, MRI has several advantages when it comes to atherosclerosis plaque imaging. First, MRI does not use ionizing radiation for imaging. Second, MRI does not require the use of a nephrotoxic contrast agent.
High-resolution MRI has been used extensively for noninvasive evaluation of the arterial walls (19,20,21). With improvements in imaging technology, the ability of magnetic resonance (MR) to delineate these vessels has significantly improved (22,23,24,25,26). Over time, a diverse array of black blood MRI techniques (27,28) have developed into practical tools for the arterial imaging and evaluation of atherosclerosis. Dedicated coils and pulse sequences have become more readily available for time-efficient multislice imaging (24,25,29,30). Black blood MRI has now become the preferred method for arterial vessel wall imaging. With this strategy, the signal from the blood is nulled, allowing for a clear depiction of the arterial wall. Most MR plaque imaging is currently being performed on a whole-body 1.5 T MR system.
The innate difference in relaxation times among various tissues can generate strong contrast on the image without the use of exogenous contrast media. To take advantage of this phenomenon, black blood multicontrast MRI can be employed with different excitation times, repetition time (TR), and echo time (TE) (21,31,32). For example, T1-weighted sequences will produce a brighter signal from tissues with short longitudinal magnetization recovery times (i.e., fat). T2-weighted sequences will produce a brighter signal from tissues with long transverse magnetization decay times (i.e., dense fibrous tissue). Proton density (PD)-weighted sequences, being a mixture of both T1 and T2 contrasts, will produce brighter signal with tissues with greater free-water content. To delineate the lumen of vessel walls, a bright blood technique such as time-of-flight (TOF) imaging is typically used.
In black blood MR, images are commonly acquired using a turbo spin echo (TSE) sequence with double inversion recovery (DIR) pulses (28) to provide good contrast between the lumen and vessel wall. DIR modules consist of two 180-degree radio frequency (RF) pulses. Magnetization in the whole volume is inverted by the first nonselective RF pulse, while magnetization in the slab of interest is subsequently restored by the second selective RF pulse (Chapter 2). The application of these two preparatory RF pulses causes spins outside the slab of interest to be inverted, with no effect on spins within the slab. Image acquisition begins following a time delay (TI) necessary for magnetization of inverted blood flowing into the imaging slice to reach null point (28). DIR techniques can be used to acquire multiple imaging slices simultaneously (25,26).
Two-dimensional (2D) and three-dimensional (3D) black blood (25,28,33) multicontrast techniques are commonly used in MR to visualize the vessel wall. To enhance the visualization of atherosclerotic plaque, intravenously administered contrast agents are used in conjunction with MR imaging (34
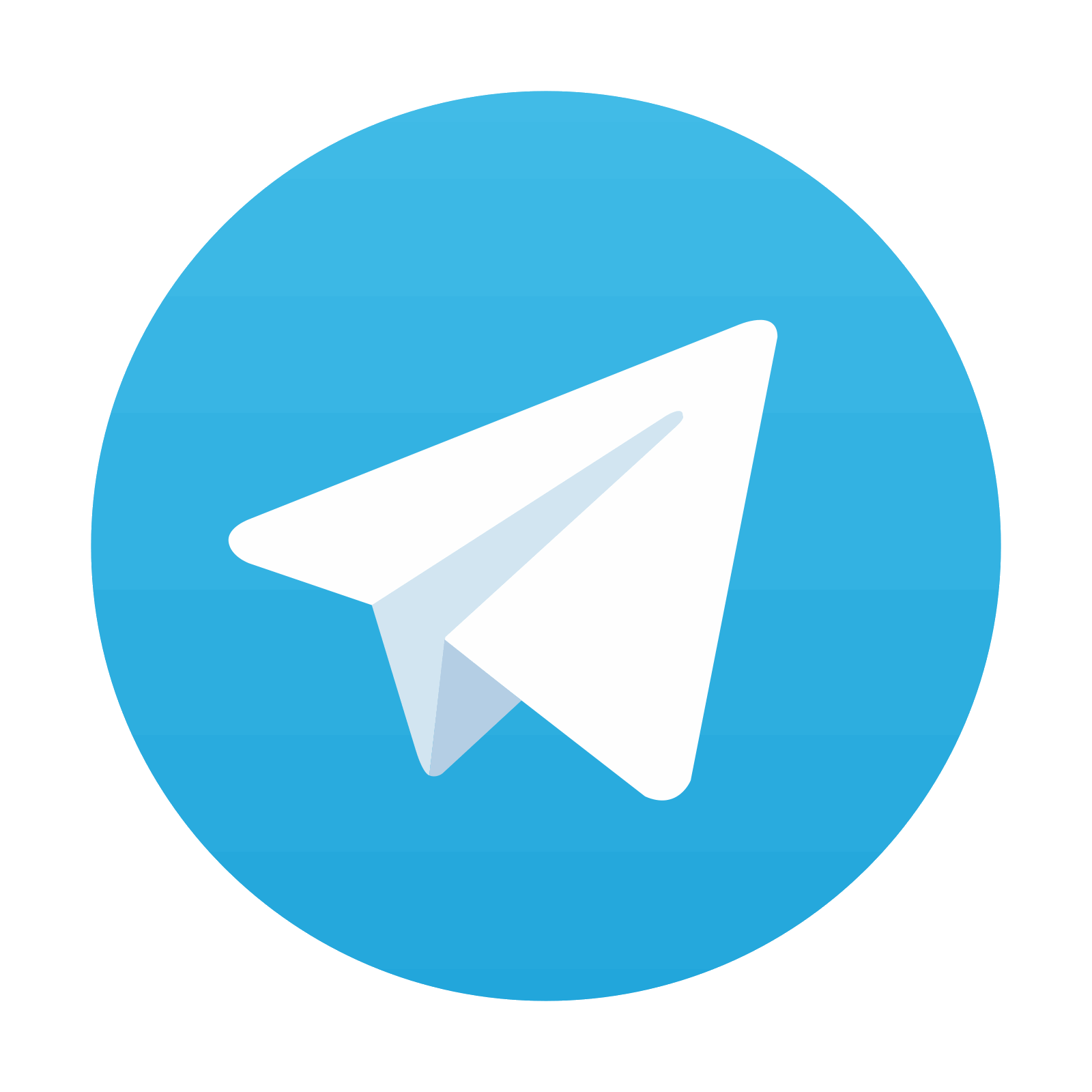
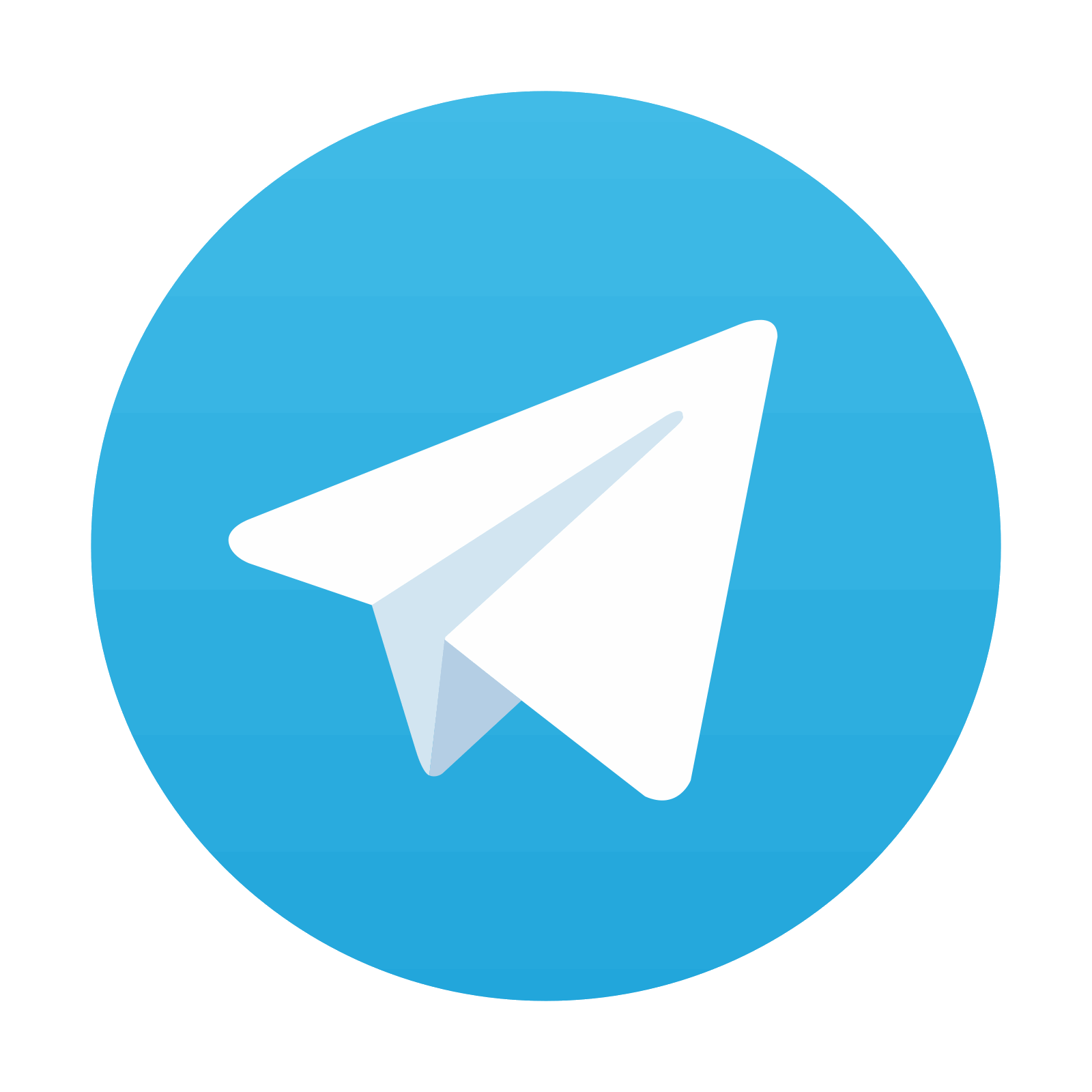
Stay updated, free articles. Join our Telegram channel
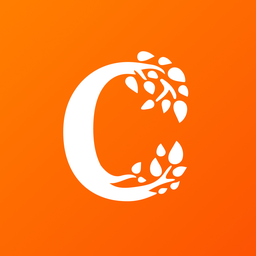
Full access? Get Clinical Tree
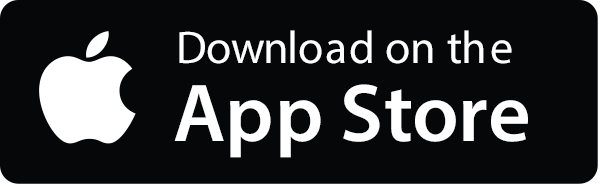
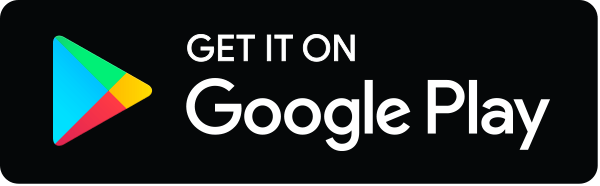