CHAPTER 23 David P. McLaughlin, Samuel S. Wu and George A. Stouffer The coronary arteries are the first vessels to branch off the aorta and through them the heart receives about 5% of the cardiac output when the body is at rest, or 250 mL/min. The heart has the highest oxygen (O2) consumption per tissue mass of all human organs. Under basal conditions, myocardium extracts approximately 75% of delivered oxygen (the myocardium has a basal metabolic requirement that is approximately 15–20 times that of resting skeletal muscle and approximately equal to that of skeletal muscle under severe acidotic conditions). The heart has the highest arterial–venous difference in O2 concentration of any major organ (10–13 mL/100 mL) and the oxygen saturation in the coronary sinus is one of the lowest in the body (Figure 23.1). Figure 23.1 Oxygen extraction in cardiac muscle. Determinants of myocardial oxygen demands include preload, afterload, heart rate, contractility, and basal metabolic rate. Other than basal metabolic rate, these are factors that influence stroke volume. Systolic wall tension uses approximately 30% of myocardial oxygen demand. Wall tension itself is affected by intraventricular pressure, afterload, end‐diastolic volume, and myocardial wall thickness. Because there is little room for increased oxygen extraction and the heart has minimal capacity for anaerobic metabolism, increased metabolic demands of the heart are met primarily via increases in coronary blood flow (i.e., flow is tightly coupled to oxygen demand). In the absence of obstructive epicardial coronary artery disease, coronary blood flow is primarily controlled by changes in resistance in the small arteries and arterioles. Resistance within the microvessels plays an important role in myocardial perfusion in general and in regional and transmural distribution. Camici and Crea describe a three‐compartment system in the coronary circulation [1]. The proximal compartment includes the large epicardial coronary arteries, which offer little resistance to coronary blood flow in the absence of obstructive disease. The intermediate compartment includes prearterioles, which offer some resistance to flow and there is a measureable pressure drop along their length. The specific function of the prearterioles is to maintain pressure at the origin of arterioles within a narrow range despite changes in perfusion pressure or flow (important in autoregulation). The more distal compartment includes intramural arterioles and there is a considerable drop in pressure. The function of the distal compartment is to match myocardial blood supply and oxygen consumption. For the purpose of understanding clinical applications of physiologic testing of coronary blood flow, the intermediate and distal compartments can be lumped together (labeled the microvasculature), creating a two‐component model. Normal epicardial coronary arteries provide little if any resistance to myocardial blood flow even at maximal flow, and are commonly referred to as conductance vessels. In contrast, the microcirculation provides the majority of resistance to flow under normal resting conditions. This chapter will not discuss microvascular dysfunction, but it is important to keep in mind that microvasculature dysfunction has been reported in patients with prior myocardial infarction and coronary artery disease risk factors [2]. Coronary blood flow can increase two‐ to fivefold in the normal heart. In one study of adult patients with angiographically normal vessels and coronary artery disease risk factors, coronary blood flow increased by 2.7 ± 0.6 times with maximal coronary vasodilation [3]. The ratio of maximal coronary flow to resting coronary blood flow is labeled coronary flow reserve (CFR). Maximal coronary blood flow can be achieved experimentally using any one of three different mechanisms. Reactive hyperemic flow is that flow that occurs following a transient period of flow cessation. Metabolites of ischemia accumulate during interruption of flow and vasodilate the microcirculation. When flow is reestablished, the resistance of the microcirculation is markedly diminished and coronary blood flow increases. Maximal coronary blood flow can also be achieved using exercise or another physiologic stimulus to increase flow. Lastly, maximal coronary flow can be achieved by intracoronary administration of potent microcirculation vasodilators such as adenosine. Coronary blood flow is primarily controlled by release of local metabolites such as adenosine or nitric oxide. Hypoxia is a more potent coronary vasodilator than either hypercapnea or acidosis. Neural influences on coronary blood flow are relatively minor. Sympathetic activation to the heart results in transient vasoconstriction, followed by coronary vasodilation due to increased metabolic activity. Parasympathetic stimulation of the heart directly stimulates coronary vasodilation, but this effect is modest. Coronary blood flow is unique in that it primarily occurs during diastole because of systolic compression of myocardial arteriolars and capillaries by the contracting myocardium. Systolic compressional forces are much greater in the subendocardial layers than in the epicardial arteries. Flow in the left coronary artery has a greater diastolic predominance than the right coronary artery because the compressive forces of the right ventricle (underlying a portion of the right coronary artery) are less than those of the left ventricle. At least 85% of coronary flow in the left anterior descending occurs in diastole, whereas the right coronary artery blood flow is more or less equal in systole and diastole. The predominance of flow during diastole exacerbates myocardial ischemia during tachycardia. With increased heart rates, oxygen supply is reduced (because diastole is shortened) while demand increases. The heart has the ability to maintain coronary blood flow in the presence of varying perfusion pressures (termed autoregulation). Autoregulation maintains consistent coronary flow over a range of perfusion pressures from 60 to 150 mm Hg (Figure 23.2), although there is evidence that autoregulation is exhausted at a higher pressure (approximately 70 mm Hg) in patients with left ventricular hypertrophy [4]. In the setting of maximum vasodilation of coronary resistance vessels, coronary blood flow is no longer autoregulated and varies linearly with perfusion pressure. One example of an autoregulatory mechanism is the Bayliss phenomenon in which increased perfusion pressure causes reflex vasoconstriction. The ability of autoregulation to maintain flow when perfusion pressures are decreased is especially important in the presence of epicardial coronary stenoses. Figure 23.2 Coronary autoregulation. Source: Polese 1991 [4]. Reproduced with permission of Wolters Kluwer.
Coronary hemodynamics
Basic principles of coronary blood flow
Regulation of coronary blood flow
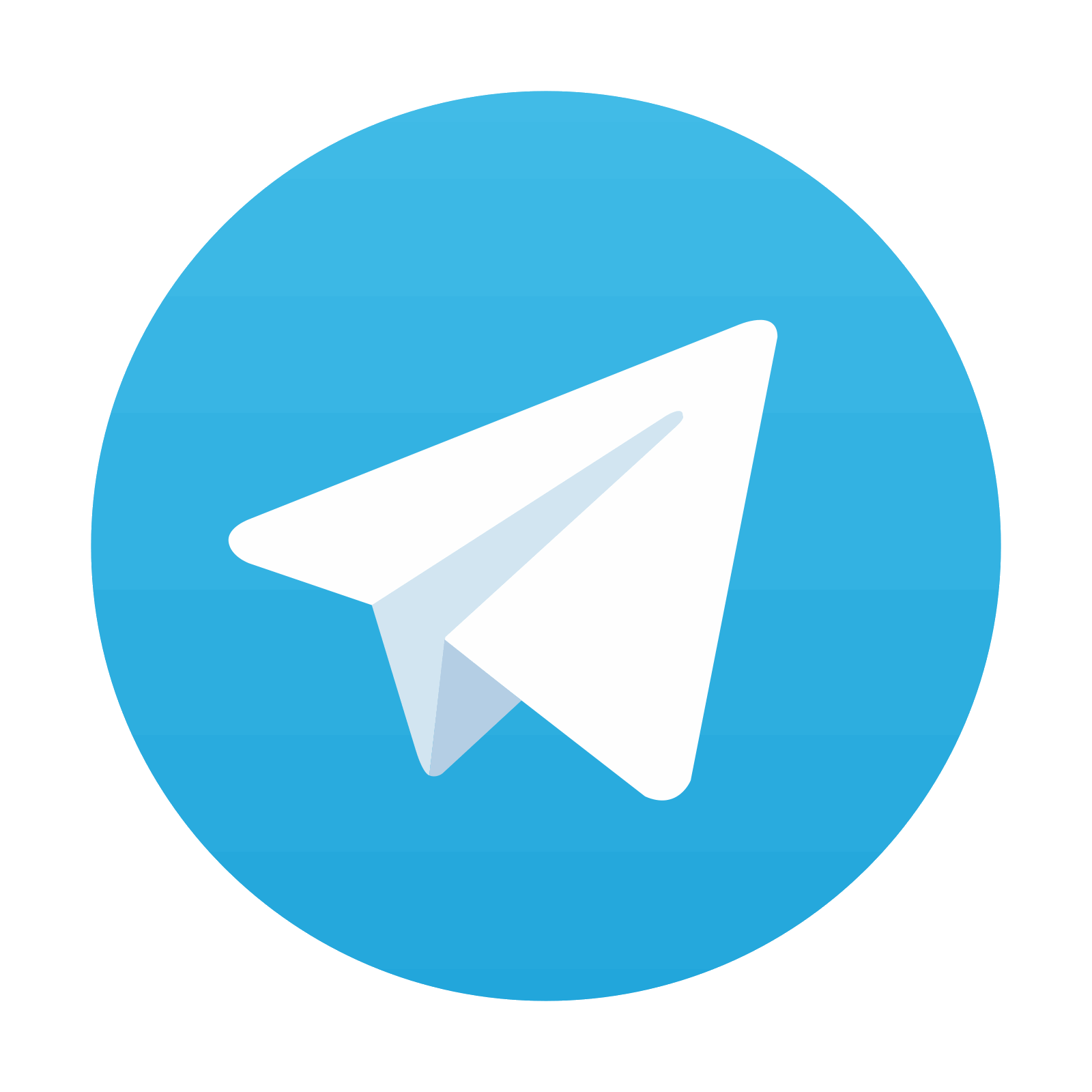
Stay updated, free articles. Join our Telegram channel
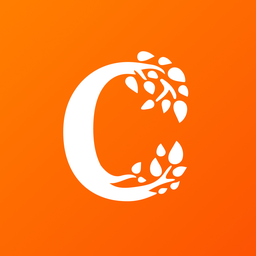
Full access? Get Clinical Tree
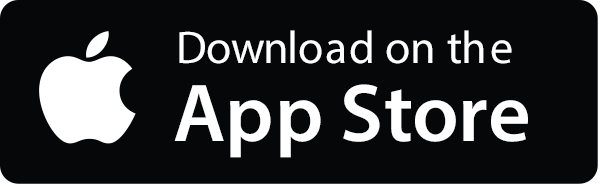
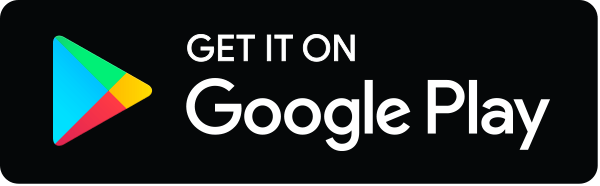