Introduction
Thoracic application of ultrasonography can be broadly divided into lung and pleural imaging. Additional applications designed for the evaluation of patients with dyspnea include vascular scanning for the diagnosis of lower limb deep venous thrombosis and basic ultrasonography of the heart. Furthermore, ultrasonography provides visual guidance for interventions in the pleural space. Instead of being considered as an optional thoracic imaging modality, bedside ultrasonography is increasingly seen as an extension of physical examination in the clinical evaluation and management of patients.
Ultrasonography continues to revolutionize pulmonary medicine by extending bedside diagnostic capabilities. Concerns remain regarding the medicolegal implications of errors in image interpretation. However, the utility of ultrasonography is gradually making the converse true as well: it is increasingly inexcusable not to use ultrasonography. Therefore it appears inevitable that ultrasonography will become established in the standard curriculum of pulmonary training.
Basics of Ultrasonography
Ultrasound Physics
Ultrasound is nonaudible sound energy used for medical applications in a frequency range of 2 to 20 MHz, frequencies that have been found to provide the best combination of penetration and resolution in the body. These frequencies are more than 1000 times greater than the audible range of sound, which ranges up to only 20 kHz. As ultrasound waves travel through tissue, they undergo attenuation and are also deflected by scattering, refraction, and reflection; it is the reflection of the waves that forms the basis of the ultrasonographic images. Reflection depends on the difference in sound transmission characteristics of the tissue, or the impedance, which is a measure of the resistance to propagation of sound waves from one medium to another. At the boundary between two tissue structures with different impedance (i.e., an impedance mismatch), an ultrasound pulse is partially reflected. The greater the difference in acoustic impedance, the greater the strength of the reflected ultrasound signal will be. The difference in impedance between air and tissue is greatest, and thus almost all energy is reflected back; a coupling gel is necessary to remove all air from between the transducer and the skin to allow ultrasound energy to enter the body. The more minor differences in impedance between different body tissues allow partial reflection of waves that impart information about the location and characteristics of the tissues. Signal processing of the reflected energy into a gray-scale image on a screen forms the basis of ultrasonographic technology. ( Table 20-1 provides a glossary of ultrasonographic terminology.)
Acoustic enhancement | An artifact showing an increase in echogenicity in a region distal to a fluid collection. |
Acoustic impedance | Defined as the ratio of sound pressure to flow. A measure of the “resistance” of the medium to the transmission of sound waves from one tissue to another. The difference in acoustic impedance (mismatch) between two tissues determines the reflection of waves at the interface. |
Acoustic shadow | Anechoic area behind bony structures. |
Acoustic window | Pathway of an ultrasound beam between the probe and the target. |
Alveolar interstitial pattern (or syndrome) | Multiple B-lines that signify the presence of thickened interlobular septa. This sign is defined by at least three B-lines in any single intercostal space and in at least two scanning zones of the thorax. |
Attenuation | The progressive reduction of amplitude and intensity of a transmitted signal as it moves through a medium. |
Artifacts | Images created by physical effects on sound wave propagation not reflecting the presence of actual tissues. |
Artifact lines | |
Comet-tail artifacts | Vertical hyperechoic lines arising from the pleural line (include B- and Z-lines). |
B-lines | Comet-tail artifacts that spread all the way to the edge of the screen without fading. |
Z-lines | Comet-tail artifacts that fade quickly without reaching the edge of the screen. |
Other Lines | |
A-lines | Horizontal hyperechoic echoes of the pleural line. |
Doppler | Technique for imaging moving particles by the detection of a change in the frequency of the reflected ultrasound energy. |
Echogenicity | |
Hyperechoic | An appearance that is brighter or more white than that of normal solid organ structures such as the liver or spleen, which are considered isoechoic. |
Hypoechoic | An appearance that is darker or more black than that of normal solid organ structures. |
Anechoic | An appearance that is black and without echoes. |
Lung sliding | The “to-and-fro” twinkling movement of the lung during respiration as it slides past the chest wall at the pleural line. |
Modes | The means by which reflected ultrasound energy is displayed on the screen. In each case, the time for the energy to be reflected from an object determines the depth of the resultant image on the screen. |
A-mode | Amplitude mode—reflected energy is shown as peaks of different size; not currently used in thoracic ultrasonography. |
B-mode | Brightness mode—reflected energy is displayed as areas of different brightness; used to generate the standard two-dimensional ultrasonographic image. |
M-mode | Motion mode—reflected energy is shown as areas of brightness traced from left to right on screen with time on the x-axis, used as an adjunct to B-mode. |
M-mode signs | |
Sinusoid sign | A hyperechoic sinusoidal line deep to a pleural effusion that represents movement of the visceral pleura with respiration adjacent to a pleural effusion. |
Seashore sign | A grainy “sandy” image with a few horizontal lines denoting the “sea” at the top, a sign that represents aerated lung. |
Stratosphere sign | A series of prominent horizontal lines that represents a pneumothorax. |
Lung point | A sudden transition from a seashore to a stratosphere sign, representing the fleeting appearance of aerated lung expanding in inspiration into a pneumothorax. A positive ultrasonographic sign for pneumothorax. |
Phased array probes | Probes that can steer ultrasound beams electronically by pulsing of transducer elements in sequence (phases). This allows a highly focused ultrasound beam to scan like a searchlight through tissue before a composite B-mode image is assembled. |
Pleural line | Horizontal hyperechoic line that corresponds to the interface between the lung and the chest wall. |
Resolution | The ability to display two tissue interfaces that are close to each other as separate images by ultrasonography. |
Time-variable gain | An amplification of reflected ultrasound energy proportional to the timing of the returning signals to enable deeper objects to be as bright as those closer. |
The ultrasound pulse is generated by the piezoelectric crystals within the transducer probe. When an alternating current is applied, these crystals vibrate to convert electrical energy into mechanical energy and produce an emitting pulse. After the pulse is transmitted, the system awaits the return of reflected signals, which are received by the same piezoelectric crystals in the transducer. Returning ultrasound energy provides two types of information: the amplitude of the signal, which indicates how much energy is reflected, and the timing of the returning signal, which is related to the distance of the target from the emitting probe.
Ultrasonographic Modes
The processing of the reflected signals determines the type of image seen. In the A-mode (or amplitude mode), the received amplitude of energy from the transducer is displayed as peaks or waves shown at the distance determined by the depth of the image. This mode is not used currently in thoracic ultrasonography. With the B-mode (or brightness mode), the amplitude of energy is instead displayed as spots of different brightness, so that a series of B-mode images can be used to produce a conventional two-dimensional ultrasonographic image. In the M-mode (or motion mode), the image of a particular object is traced by capturing a B-mode image as it sweeps across the screen against a time axis. This provides a single image in which relative positions of images are shown against time on the x-axis. The B-mode is the standard mode for all studies, and the M-mode is usually used as an adjunct, especially when B-mode findings are equivocal.
Transducers
Thoracic ultrasonography typically uses the 3.5- to 5-MHz frequency range, which provides the optimal compromise between spatial resolution and depth of penetration for thoracic indications. Higher frequencies improve resolution but limit the depth of imaging; lower frequencies visualize with less resolution but to a greater depth. Thoracic ultrasonography generally uses curvilinear, or microconvex, probes, which employ a curved surface to create a scanned field that is wider than the footprint of the probe, producing a sector or fan-shaped image; small footprints can also be achieved with phased array probes with electronic steering. Small footprints enable better access through the narrow acoustic windows available between ribs through which the beam can access deeper tissues. Compared to linear probes, curvilinear transducer probes have reduced lateral resolution; however, the resolution is acceptable for discriminating the relative sizes of structures imaged in the chest.
Doppler Ultrasonography
Doppler imaging is used to detect motion. Doppler imaging is based on the principle that the frequency of sound increases as the source of sound moves toward an observer and decreases as the source of sound moves away. In pulsed wave Doppler, a pulse is transmitted and the change in frequency of the returning echo is measured at a certain time. Traditionally, red denotes objects moving toward the probe and blue on the screen denotes objects moving away from the probe. Optimal imaging for color Doppler is obtained when the transducer is directed parallel to the flow of the target; in contrast, for B-mode and M-mode ultrasonography, optimal imaging is produced when the transducer is directed perpendicular to the target.
Ultrasonographic Image Acquisition
Positioning of Patients and Probe
In a free pleural space without septations, effusions will collect in the dependent parts of the thorax, whereas free air will collect in superior, nondependent locations. Therefore the positions of the patient and ultrasonographic probe are crucially important to evaluating these indications. In most thoracic evaluations the patient is examined sitting upright. Patients who are ill may be examined in the supine or lateral decubitus position, but this must be taken into consideration when images are interpreted. By abducting the arm, the intercostal space distance can be increased to provide a larger acoustic window. For a systematic evaluation, the hemithorax should be examined in each of the four zones demarcated by the parasternal, anterior axillary, and posterior axillary line ( Fig. 20-1 ). The patient is then asked to sit upright and is scanned along the posterior paravertebral line. Every scan must visualize the diaphragm inferiorly. For better access to the posterior thorax, supine patients who cannot sit up can be moved to the lateral edge of the bed.

Scan/Probe Orientation
Longitudinal scans through the intercostal spaces (i.e., parallel to the long axis of the body) are preferred to transverse scanning both because of convention and because a longitudinal image will include the diaphragm for ease of reference. To maintain orientation of the probe and the resulting image, the transducer probe has a ridge or groove. With the groove oriented toward the head of the patient, the resultant image will show the cranial direction to the left of the screen. Superficial structures will be at the upper part of the screen and deep structures at the bottom of the screen ( Fig. 20-2 ).

Probe Handling
The probe is held in the dominant hand as one would hold a pen, with the forefinger on the orientation groove. The examiner’s hand rests against the patient’s skin as a support to stabilize the transducer. The probe is moved from intercostal space to intercostal space and moved transversely within intercostal spaces. For this movement, called “transducer movement,” the probe is held perpendicular to the skin. For two other movements, the probe is tilted: “transducer tilt” describes the rocking movement of the probe at any particular position to obtain images along the same tomographic plane and “transducer angulation” to obtain images in adjacent planes. Tilt and angulation are fine movements, and too much of either can result in loss of contact with the skin, causing a poor image. Because air forms an almost impermeable barrier to ultrasound, air trapping is minimized by using a water-based coupling gel and by holding the probe firmly against the skin. The probe must be kept still during image acquisition to prevent confusion between the movement of the probe and dynamic signs within the thorax.
Image Acquisition
Two parameters need to be adjusted for optimal acquisition of the images: depth and gain. The depth of the field is set according to the needs of the ultrasonographic examination: a 10-cm depth will usually suffice for most thoracic investigations. Less depth magnifies the image and allows clearer visualization of the near field, as, for example, for evaluating the pleural surface for lung sliding. Deeper penetration may sometimes be needed to define boundaries of the image of interest. The gain is set to amplify signals relative to background noise. Increasing the gain makes an image whiter, whereas decreasing the gain makes the image blacker. Time-variable gain enables the operator to amplify ultrasound signals proportional to the depth to make deeper structures appear as bright as more superficial structures. Because solid organs such as the normal liver are considered to be of intermediate density (isoechoic), gain can be adjusted with reference to the liver image to make it gray on the screen; other tissues can then be evaluated in comparison to this standard gray image. Although noise filters can improve the quality of imaging solid and liquid structures, the use of such filters can also obliterate the signal artifacts that are crucial to diagnostic analysis of aerated lung.
Image Interpretation
Two types of images are visualized by ultrasonography, either anatomic structures or artifacts. Anatomic structures are identified based on their location and echogenicity. Echogenicity of body structures can be referenced to solid organs such as the liver, which are made to appear gray or isoechoic on the screen. Uncomplicated fluid collections appear darker than these solid organs and are termed “hypoechoic”; if they appear black, they are termed “anechoic.” Air appears white and is hyperechoic. The homogeneity or heterogeneity of echoes also contributes to the recognition of different tissues.
Artifacts are images that do not correspond to specific anatomic structures but provide useful information if properly recognized. Examples of artifacts are acoustic shadows, acoustic enhancement, and reverberation echoes (see Table 20-1 ). Unlike anatomic structures, artifacts tend to move with the movement of the probe and converge toward the near field at the top of the screen. Acoustic shadows are anechoic regions that lie behind bony structures such as ribs. Because acoustic shadows provide no ultrasound information, the transducer probe should have an appropriately small footprint to transmit ultrasound pulses between the bony structures and thus avoid them. Acoustic enhancement is a hyperechoic region (lighter than liver) located distal to a fluid collection; the enhanced region distal to the fluid should not be mistaken for another tissue structure. Reverberation echoes are alternating dark and white lines produced when ultrasound beams reflect back and forth between two surfaces, often at an air–soft tissue interface. These echoes produce multiple-copy images seen at different depths depending on the number of times the beam was reflected ( Fig. 20-3 ).

Lung Ultrasonography
Competence in lung ultrasonography involves knowledge of terminology of ultrasound signatures in the thorax, as well as identification of consolidated lung and air artifacts. ( Table 20-2 lists lung ultrasonographic signs, and Table 20-3 compares lung and pleural ultrasonographic competencies.) Key diagnoses that can be made include pneumothorax, pulmonary consolidation, and pulmonary edema.
Condition | Ultrasonographic Findings |
---|---|
Normal lungs |
|
Pneumothorax |
|
Lung consolidation/atelectasis “Alveolar pattern” |
|
Pulmonary edema |
|
ARDS/pulmonary fibrosis “Alveolar interstitial pattern” |
|
Diaphragm paresis or paralysis |
|
Pulmonary embolism |
|
Lung Ultrasonography | Pleural Ultrasonography |
---|---|
|
|
Normal Lungs
“Lung sliding” is the key sign used to identify normal lungs. To identify lung sliding, one first identifies the “pleural line” as a horizontal hyperechoic line located approximately 0.5 cm below the skin surface corresponding to the interface between the lung and the chest wall. The pleural line is framed by anechoic shadows caused by the ribs. A-lines are deeper horizontal lines that are reverberation echo artifacts of the pleural line (see Fig. 20-3 ). Once these lines are identified, lung sliding is seen as the to-and-fro twinkling and movement of the lung adjacent to the pleural line in rhythm with inspiration and expiration ( ). The image above the pleural line on the screen is motionless. Therefore lung sliding identifies visceral pleura sliding over parietal pleura. An M-mode image of this view will show up as a grainy pattern that recedes distally and is termed the “seashore sign” ( Fig. 20-4A ).

Lung sliding is an important sign for identifying normal lung, but it is dependent on technical issues and interpretation. For example, the sign may not be detected with low-frequency probes below 2.5 MHz or if the depth is set too deep. In addition, the amplitude of lung sliding will decrease at the apex of the lung, where movement of the lung is less. Lung sliding can be mimicked by accessory respiratory muscle contractions, although, unlike lung sliding, muscle movement will appear superficial to the pleural line. Lung sliding can also be absent in pathologic conditions in which lung motion is impaired, such as pleurisy, pleurodesis, pneumothorax, subcutaneous emphysema, apnea, jet ventilation, extreme bronchospasm, extensive pneumonia, and the acute respiratory distress syndrome (ARDS). Thus the absence of lung sliding may not be useful, whereas its presence is effective in ruling out many of the abnormalities of the lung.
Pneumothorax
Ultrasonography is more accurate than supine anteroposterior chest radiographs in the diagnosis of pneumothorax. Pneumothorax itself has few positive findings; it is generally identified by the absence of the signs of normal aerated lung. As described earlier, aerated lung is noted by the presence of the lung sliding sign; the lung is also noted by artifacts called “comet tails,” including B-lines and Z-lines, which are vertical hyperechoic lines that arise from the pleural line and move in synchrony with lung sliding. B-lines spread from the pleural line all the way to the edge of the screen without fading. These lines are the most important of the comet tails, and their presence or absence can help guide the diagnostic value of ultrasonography ( ). Z-lines are similar but, unlike B-lines, fade quickly and do not reach the periphery of the screen.
In any ultrasonographic examination for pneumothorax, the nondependent part of the hemithorax needs to be scanned. In a pneumothorax, A-lines representing reverberation echoes of the pleural line will still be seen, showing that an air-tissue interface is present, but there will be no movement of the lung sliding at the pleural line and no evidence of B-lines ( ). In the appropriate clinical context, the absence of lung sliding and B-lines indicates the presence of pneumothorax with a reported 100% sensitivity and 96.5% specificity. On the other hand, the presence of the lung sliding sign basically excludes a pneumothorax, with a reported 100% negative predictive value. In a pneumothorax, multiple accentuated A-lines may be seen, and this can be captured on M-mode as a series of stratosphere-like horizontal lines (i.e., the stratosphere sign) ( Fig. 20-4B ). The horizontal lines seen in the stratosphere sign, which are characteristic of free air, can be contrasted with the grainy pattern prominent in the seashore sign of normal aerated lung (see Fig. 20-4A ). A pneumothorax can be grossly quantified as large if the loss of both lung sliding and comet tails extends to dependent parts of the thorax. The sudden and fleeting appearance of lung sliding and B-lines in an area where they were previously absent may indicate aerated lung expanding intermittently into a moderate-sized pneumothorax. This fleeting appearance if captured on M-mode is termed the “lung point” and has been reported to have 66% sensitivity and 100% specificity for the diagnosis of a pneumothorax. These ultrasonographic signs when used in combination may diagnose occult pneumothoraces not detected on supine chest radiographs and are useful in the evaluation of patients after procedures such as central venous catheter placement, transbronchial lung biopsy, and chest tube clamping. The presence of a pneumothorax must be strongly suspected if B-lines that were present before the procedure are not detected after the procedure.
Errors that can lead to a failure to diagnose pneumothorax include failure to scan in the nondependent parts of the thorax, failing to scan longitudinally, excessive use of noise filters, and an unsteady scanning hand that may give the false impression of lung sliding. A false diagnosis of pneumothorax may arise in patients with chronic obstructive pulmonary disease (COPD), lung bullae, and adhesions, which may mimic pneumothorax on ultrasonography. In patients with COPD the specificity of the diagnosis of pneumothorax is reduced to 71% even among experienced operators, indicating that additional imaging studies are required to confirm a pneumothorax in such patients.
Lung Consolidation/Atelectasis
Alveolar consolidation can be identified on ultrasonography if the area of consolidation extends to the visceral pleura; if not, the intervening aerated lung will generate artifacts that make the diagnosis difficult. In alveolar consolidation, lung sliding will be abolished and a tissue pattern similar to the liver will develop because of the consolidation and “hepatization” of the lung. This sign, which has been termed the “alveolar syndrome” in some studies and is here referred to as the “alveolar pattern,” can be due to a variety of causes, including pneumonia, atelectasis, contusion, malignancy, and infarction. Discrimination between these diagnoses may be attempted with further ultrasonographic analysis, including assessment of the deep margins of consolidation, identifying air or fluid bronchograms, and evaluating the vascular pattern within the consolidation using color Doppler.
In patients who have pneumonia, the superficial boundary of consolidated lung is usually regular and conforms to the visceral pleura, whereas the deep border is irregular and faded and varies with respiration. Comet-tail artifacts may also be seen at this far-field border. Air bronchograms may be seen as hyperechoic (white) branched or linear shadows that may vary with respiration ( Fig. 20-5 ). Air trapped in the distal airways shows up as punctate white spots. On color Doppler, branching pulmonary blood vessels may be visualized within the area of consolidation. These signs together with the absence of a sinusoidal pattern on M-mode that would indicate lung motion give ultrasonography a diagnostic sensitivity of 90% and specificity of 98% in detecting consolidation when compared to computed tomography (CT) scans. A false-positive diagnosis of consolidation may result when complex echogenic pleural effusions or intrathoracic fat are mistaken for consolidation. The size of consolidation is typically smaller on ultrasonographic images than on chest radiographs because the peripheries of a pneumonic area are usually partially air filled.
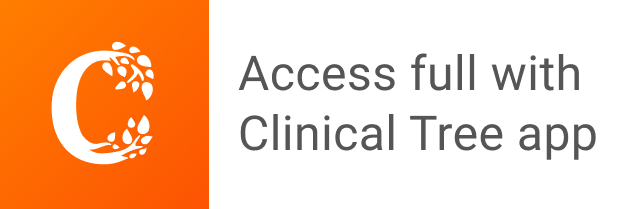