Introduction
The immune system is broadly conceptualized as having two separate but interconnected arms. In evolutionary terms the innate immune arm is an older and more primitive system that has developed to provide early host defense against viruses, fungi, and bacteria. The fundamental basis of innate immunity is a system for pathogen detection that relies on the recognition of a range of pathogen-associated molecular patterns (PAMPs), which include complex lipids, carbohydrates, unmethylated cytosine-guanosine DNA sequences, and double-stranded RNAs. PAMPs are recognized by a series of secreted, cell surface, and intracellular pattern recognition receptors (PRRs) that promote the recognition, phagocytosis, and killing of many microbes. In addition, recognition of PAMPs by PRRs initiates inflammation, which in turn leads to the recruitment of phagocytes that kill and degrade microbes, promote the repair of damaged tissues, and assist in the restoration of tissue function. Though some organisms, for example, sea urchins, have evolved a system of host defense based exclusively on the innate immune system, mammals and higher animals have evolved an adaptive immune system that differs from the innate immune system in its exquisite antigenic specificity and the ability to develop immunologic memory, allowing a more rapid response to previously encountered microbes and antigens. Both the innate and adaptive immune systems operate together and exist in a classic symbiotic relationship to provide optimal defense of the lung and other organs and tissues (see Chapter 13 ).
The broad concepts of innate and adaptive immunity outlined previously have evolved to protect most organs and tissues from microbes, a term we use to include bacteria, yeasts, fungi, protozoa, multicellular parasites, and viruses. However, most organs and tissues, including the lung, have evolved additional mechanisms to tailor the immune system to their own specific needs. The lung epithelium has a surface area approximately the size of a tennis court and represents the largest epithelial surface in the body. With an average respiratory rate of 10–12 breaths/min and an average tidal volume of 600 mL, the lungs are exposed to over 10,000 L of ambient air per day. The air that we breathe is a complex mixture of gases and particulates that can contain pollutants, oxidants, inorganic and organic dusts, pollens, toxins, bacteria and their constituents (e.g., bacterial endotoxin, lipopolysaccharides [LPSs]), and viruses. In addition, the airways and gas-exchange surfaces of the lung can be exposed by aspiration to acidic gastric contents as well as to infected mucus from the nasal sinuses. Thus all surfaces of the respiratory tract from the nasal passages to the alveoli are constantly exposed to a spectrum of harmless, harmful, and pathogenic agents, raising the key question of how the lung differentiates between what is harmful and what is essentially harmless.
Like the gut, the lung has evolved discriminative mechanisms both to suppress unwanted and potentially harmful responses to harmless materials and to retain the ability to activate a vigorous innate and adaptive immunity when encountering harmful microbes and stressors. The overall goal of this chapter is to review current concepts of lung innate immunity and its fundamental underlying mechanisms. Multiple cell lineages participate in innate protection of the lung, and many share similarities in the way they recognize and respond to microbes and other harmful agents. Therefore we have organized the chapter into four broad sections. First, we provide an overview of lung innate immunity and its fundamental components. Second, we discuss general mechanisms of innate immune recognition. Third, we review innate lung cells and the effector mechanisms they use. Fourth, we discuss how these systems and mechanisms are integrated. Throughout the chapter we also discuss how the innate immune system primes the adaptive immune system as a prelude to the chapter on adaptive immunity (see Chapter 13 ).
Overview of the Components of Lung Innate Immunity
Microbial species can rarely be cultured from the lungs of healthy individuals, leading to the long-standing dogma that the lower airways and gas exchange surfaces of the lungs of healthy subjects are sterile. However, recent studies based on culture-independent methodologies, especially bacterial 16S ribosomal RNA sequencing, have begun to challenge this notion. Based on an earlier single-center study with a modest number of subjects, a recent National Heart, Lung, and Blood Institute–sponsored multicenter study with 64 subjects has provided data to indicate that bacterial DNA is present in the bronchoalveolar lavage of healthy subjects and that most sequences resemble the diversity seen in the mouth. However, sequences from Enterobacteriaceae , Haemophilus, Methylobacterium , and Ralstonia species were disproportionately represented in the lung. Although it is unclear if these lung enriched sequences are derived from bacterial communities colonizing the throat, nasal passages, or gastrointestinal tract (which were not sampled for comparison) or whether or not they were derived from viable bacteria, these findings provide hints that commensal bacterial communities may exist in the lungs of healthy individuals. Thus, it will become increasingly important to integrate emerging information on lung microbiota into our understanding of how the innate immune system interfaces with these potentially commensal bacteria.
Given this caveat, the innate immune system is currently conceptualized as providing protection to the lungs against a diverse spectrum of inhaled particles and antigens that range from harmless nonmicrobial particles (e.g., dusts and pollens) to harmful pathogenic microbes (e.g., Mycobacterium tuberculosis ) . To accomplish the range of protection, the lungs have evolved multiple mechanisms to survey and respond to inhaled particles based primarily on their size and physicochemical properties, especially the presence and absence of PAMPs. As illustrated in Figure 12-1 , innate immune protection in the lung can be broadly divided into considerations of the conducting airways and the gas-exchange surfaces of the alveoli. At the cellular level, innate protection is afforded by the coordinated functions of airway and alveolar epithelial cells, resident macrophages and dendritic cells (DCs), and recruited neutrophils ( polymorphonuclear leukocytes [PMNs]), monocytes, and DC precursors.

Though often underestimated, an important component of airway host defense resides in the anatomic structure and epithelial cell lineages of the tracheobronchial tree. Air turbulence created by the nasal passages and the cartilaginous segmentation of the trachea and large airways ensures that particles in excess of 10 µm in diameter are deposited on the mucus-coated surfaces of the nose, pharynx, trachea, and descending airways. In turn, mucus, its ensnared particulates, and dissolved solutes are constantly wafted toward the pharynx by the coordinated beating of ciliated airway epithelial cells where their removal is aided by coughing, sneezing, and swallowing. In addition to the biophysical properties of mucus in innate host defense of the airways, the gel and pericellular liquid phases also contain an array of antimicrobial peptides and proteins, antioxidants, antiproteases, and specific immunoglobulin A (IgA) antibodies ( Table 12-1 ), all of which are maintained at a modestly acidic pH (pH 6.6). Principal among airway antimicrobial peptides in humans are salt-sensitive cysteine-rich cationic β-defensins and the cathelicidin LL37/hCAP18. β-defensin-1 is constitutively secreted by airway epithelia and accumulates in airway surface liquid at microgram per milliliter concentrations. Other β-defensins and LL37/hCAP18 are inducibly expressed following exposure to LPS and other proinflammatory mediators. Antimicrobial proteins, including lactoferrin and lysozyme, are also present in airway epithelial secretions and contribute to the maintenance of normal airway sterility.
Lysozyme |
Lactoferrin |
Secretory leukoprotease inhibitor |
Uric acid |
Peroxidase |
Aminopeptidases |
Statherin |
Secretory phospholipase A 2 |
β-defensins |
Natural IgA antibodies |
SP-A * |
SP-D * |
The conducting airways are lined with ciliated and secretory epithelial cells that serve a key role in the initial evaluation of the surfaces of large particles with which they come into contact. In addition, a network of DCs resides throughout the airway epithelium and continuously samples the airway lumen (see Fig. 12-1 ). DCs are particularly abundant in the trachea and large conducting airways where most large particulates are deposited. In the absence of PAMPs, DCs capture airway antigens and migrate to regional lymph nodes where, for harmless antigens, they induce CD4 + and CD8 + T-cell tolerance.
In contrast to large particles, particles smaller than 5 µm in diameter are able to descend the entire tracheobronchial tree and lodge at bronchiolar-respiratory duct junctions or deposit onto the surfactant-rich surfaces of the alveoli. While sharing many similarities with innate protection of the airways—for example, the presence of antioxidants, antiproteases, and antimicrobial enzymes—additional innate protection of the alveoli is afforded by the presence of resident alveolar macrophages and the lung-specific collectins, surfactant protein A (SP-A) and surfactant protein D (SP-D) (see Fig. 12-1 ). In addition to expressing Toll-like receptors (TLRs), resident alveolar macrophages express scavenger receptors (SRs) that participate in the phagocytosis of microbial and nonmicrobial particles. SP-A and SP-D are secreted PRRs and are capable of binding to microbial PAMPs, leading to opsonization and phagocytosis by alveolar macrophages and intraseptal DCs, which then either crawl into the airways and move up the mucociliary escalator or migrate to regional lymph nodes, respectively. In the absence of PAMPs, resident alveolar macrophages also play an important role in suppressing inflammation and adaptive immunity, thereby protecting the alveoli from unwanted responses to harmless inhaled particulates. SP-A and SP-D also play a key role in suppressing inflammation in the absence of PAMPs through tonic signaling effects on resident alveolar macrophages. Thus the innate immune system not only protects the lungs from harmful microbes, but also prevents inflammation, injury, and activation of the adaptive immune system in the steady state and in response to harmless inhaled particulates.
How then, do the airways and alveoli respond to the presence of potentially harmful microbes? As illustrated in Figure 12-1 , resident airway and alveolar macrophages, DCs, and airway and alveolar epithelial cells are capable of recognizing different PAMPs through their repertoires of cell surface and intracellular PRRs, and by the interaction of PAMP-bound secreted PRRs with specific receptors on epithelial cells, macrophages, and DCs. In turn, these interactions induce signaling responses that promote the expression of an array of innate response genes ( Table 12-2 ). These gene products collectively promote the migration of PMNs and monocytes from the pulmonary circulation into the air spaces, facilitate changes in endothelial and epithelial permeability to enhance inflammatory cell transmigration into the air spaces, and initiate the expression of specific genes involved in microbial killing. In addition, DCs phagocytose PAMP-expressing microbes, mature, and migrate to regional lymph nodes (see Fig. 12-1 ). During this process, ingested microbial products are digested, captured by major histocompatibility complex (MHC) class II molecules, and displayed on the DC plasma membrane together with co-stimulatory molecules such as CD40, CD80, and CD86 for effective presentation to naive CD4 + and CD8 + T cells. Following activation and expansion in regional lymph nodes, effector CD4 + and CD8 + T cells then migrate back to the site of microbial infection to augment specific host defense through their ability to activate macrophages and other effector cells.
CXCL CHEMOKINES |
CXCL1 , CXCL2 , CXCL4 , CXCL9 , CXCL10 , CXCL11 |
CCL CHEMOKINES |
CCL1 , CCL2 , CCL7 |
CYTOKINES |
TNF-α , IL-1β , TGF-β , IL-10 |
TLRS |
TLR2 , TLR4 , TLR9 |
PROSURVIVAL |
BCL2 , cIAP1 , cIAP2 , BCL10 |
ANTIMICROBIAL |
β-defensins, cathelicidins |
DC MATURATION |
CD40 , CD80 , CD86 , MHC Class II, Class II |
In summary, innate immune mechanisms involving the airway and alveolar epithelium, secreted antimicrobial enzymes, PRRs and peptides, mucus and mucociliary transport, and resident macrophages and DCs protect the lung against inhaled microbial and nonmicrobial particulates to maintain lung homeostasis. During steady-state conditions, the innate immune system actively suppresses inflammation and promotes tolerance to commonly inhaled harmless particulates. However, above a certain threshold and/or upon sensing the presence of PAMPs, additional mechanisms are activated to protect the lungs by promoting inflammation and adaptive immunity and by establishing communication and cooperation between these systems. Although it is convenient to think of these events separately, innate host responses to both harmless nonmicrobial particulates and harmful microbes take place simultaneously and silently to maximize lung health and protection.
Innate Recognition in the Lung
With this broad overview of the key elements in innate host defense of the lung and their connections to adaptive immunity, we now consider how microbes are recognized by resident and recruited lung cells. For this purpose, we specifically focus on the mechanisms of recognition by PRRs that are expressed by epithelial cells, macrophages, and DCs or are present in airway or alveolar surface liquids.
Secreted Pattern Recognition Receptors
Secreted PRRs have evolved to serve as bridges between certain PAMPs and specific receptors for these molecules. In the lung, secreted PRRs have particularly important roles in innate protection of the alveolar surfaces.
Collectins
The collectins are a family of secreted PRRs; SP-A and SP-D are collectins that are uniquely expressed in the distal lung. All members of the collectin family are characterized by the presence of a cysteine-rich N-terminal noncollagenous domain, a collagen-like domain, an α-helical coiled-coil neck domain, and a globular C-type lectin domain (also called the “ carbohydrate recognition domain ” [CRD]) that interacts with microbial PAMPs ( Fig. 12-2A ). At baseline, SP-A and SP-D suppress inflammation (see later) (shown for SP-D in Fig. 12-2B ); in the presence of a variety of PAMPs, SP-A and SP-D stimulate microbial phagocytosis, the production of reactive oxidant species, and the expression of proinflammatory cytokines (see Fig. 12-2C ). A proinflammatory response is activated when PAMP-bound SP-A and SP-D interact with macrophages via their collagenous tails through CD91 (see Fig. 12-2C ). Consistent with these findings, SP-A-deficient and SP-D-deficient mice have increased susceptibility to pulmonary infection with Pseudomonas aeruginosa and Staphylococcus aureus , emphasizing the important contribution of these lung-specific collectins to innate lung host defense. Additional information on SP-A and SP-D can be found in Chapter 8 .

The lung is remarkable in that it is capable of eliminating harmful pathogens from the alveolar surfaces while actively suppressing unwanted and potentially harmful inflammatory responses to harmless inhaled materials. Several studies have shed new light on this dichotomy by revealing an additional role for SP-A and SP-D in the tonic suppression of lung inflammation. When initially created, SP-D-deficient mice were found to have increased numbers of foamy, activated alveolar macrophages, suggesting that SP-D may somehow tonically suppress lung macrophage activation and lung inflammation (see Fig. 12-2B ). As illustrated in Figure 12-2C , in the steady state (i.e., in the absence of PAMPs), SP-D and SP-A interact with alveolar macrophages via their globular head groups and signal via signal-inhibitory regulatory protein α to suppress proinflammatory responses. Thus SP-A and SP-D, through their ability to interact under different circumstances with CD91 and signal-inhibitory regulatory protein α, play a pivotal role in the maintenance of the antiinflammatory environment of the alveoli under steady-state conditions, while promoting an inflammatory and innate response when microbes are sensed. Other studies suggest that lipid and phospholipid constituents of surfactant also contribute to maintenance of the antiinflammatory environment of the alveoli.
Complement
The complement system functions as a pattern recognition system and a bridge to adaptive immunity through its ability to recognize repetitive structures on some microbes and on the Fc region of immunoglobulin G (IgG) and immunoglobulin M (IgM) antibodies. Complement activation is important in lung innate immunity because it promotes the opsonization of microbes and the generation of the potent chemotactic factor C5a, which in turn assists in the recruitment of phagocytic cells. Complement components of the classical, alternative, and mannose-binding lectin activation pathways are present in lung airway and alveolar fluids and are synthesized by alveolar type II cells, macrophages, and DCs. Many microbes can directly activate the alternative complement pathway, resulting in the covalent attachment of C3b to the microbial cell wall. Microbes expressing cell wall–associated mannose-rich polysaccharides can also bind mannose-binding lectin and activate the mannose-binding lectin pathway to promote C3b attachment to the microbial cell wall. Phagocytic cells, especially macrophages, PMNs, and DCs, express various receptors for C3b and C3bi and, together with other receptors, phagocytose and kill complement-opsonized microbes.
Pentraxins and Other Secreted Pattern Recognition Receptors
The pentraxins, including C-reactive protein, serum amyloid P, and pentraxin 3, also recognize PAMPs on microbes and activate the complement system to assist in microbial removal by phagocytic cells. Similarly, ficolins promote phagocytosis and complement activation by binding to the gram-positive bacterial cell wall components N -acetylglucosamine and lipoteichoic acid.
Cellular Pattern Recognition Receptors
Different families of PRRs have evolved to enable the host to sense the presence of PAMPs in extracellular, endosomal, and cytoplasmic compartments, in each of the lineages involved in lung innate immunity ( Fig. 12-3 ). Transmembrane cell surface and endosome-associated PRRs include the TLRs widely expressed on epithelial cells, macrophages, PMNs, and DCs (see Fig. 12-3A ), SRs primarily expressed on macrophages (see Fig. 12-3B ), and C-type lectin receptor s mainly expressed on DCs (see Fig. 12-3C ). Cytoplasmic PRRs are composed of nucleotide-binding and oligomerization domain (NOD) –like receptors (NLRs) and RNA helicases of the retinoic acid–inducible gene (RIG) family. Each of the cytoplasmic PRRs has evolved as a strategy to alert the host to the presence of PAMPs within the cytoplasm, as often happens during the intracellular replication of facultative bacteria and viruses.

Plasma Membrane and Endosomal Pattern Recognition Receptors
Toll-like Receptors.
TLRs are expressed on airway and alveolar epithelial cells, macrophages, PMNs, and DCs. Thirteen TLRs have been described in mice (Tlr1 to Tlr13) and 10 in humans. Significant progress has been made in understanding the functions and downstream signal transduction pathways of TLRs. TLRs can be divided into those expressed on the cell surface (TLR1, 2, 4, 5, 6, and 11) and those expressed in intracellular compartments (TLR3, 7, 8, and 9) (see Fig. 12-3A ). TLRs also recognize endogenous ligands called danger-associated molecular patterns (DAMPs), including heat shock proteins, low-molecular-weight hyaluronan, heparin sulfate, fibronectin, high-mobility-group box-1, protein and low-density lipoproteins. Recognition of DAMPs by TLRs and other PRRs is usually associated with the initiation of sterile inflammation. Surface TLRs recognize a wide variety of PAMPs, whereas intracellular TLRs mainly recognize nucleic acid–based PAMPs. TLRs not only recognize their individual cognate PAMPs but also combine with other TLRs to form heteromeric complexes that recognize a broader range of PAMPs. For example, TLR1 associates with TLR2 to recognize microbial lipopeptides, TLR2 and TLR4 recognize the LPS-binding protein/myeloid differentiation protein-2 complex when presented by CD14, and TLR2 recognizes broad patterns of lipoproteins in concert with either TLR1 or TLR6. TLR5 recognizes flagellin and is particularly important in the response of airway epithelial cells to P. aeruginosa infection. Similarly, TLR6 can functionally associate with TLR2 to recognize a variety of microbial lipopeptides and peptidoglycans from gram-positive bacterial cell walls. TLR3, 7, 8, and 9 are not highly expressed at the cell surface but are expressed intracellularly in endosomal and endoplasmic reticulum membranes, where they sense nucleic acids that are released from ingested and digested microbes. TLR3 recognizes viral double-stranded RNA and induces the production of type I interferons (IFNs) (IFN-α/IFN-β), which in turn play a vital role in antiviral immunity and in DC maturation. TLR7 recognizes single-stranded RNA from viruses, whereas TLR9 recognizes unmethylated cytosine-guanosine motifs in microbial DNA.
Scavenger Receptors.
The first macrophage SR was described by Goldstein and colleagues and Brown and associates and was shown to bind and internalize acetylated low-density lipoprotein. Since then, multiple SRs have been identified and shown to play diverse roles in the phagocytosis of a variety of particles and molecules ranging from bacteria to lipids (see Fig. 12-3B ). The SR family comprises eight classes (A to H), of which class A (SRA) and class B (SRB) are primarily involved in innate immunity through their ability to recognize and promote phagocytosis of a wide range of bacteria. Members of the SR-A and SR-B subfamilies are abundantly expressed on macrophages but do not appear to be expressed by airway or alveolar epithelial cells. The lack of expression on epithelial cells is in keeping with the notion that, during exposure to inhaled microbes and particles, the lung epithelium has evolved mechanisms to respond to PAMPs by promoting inflammation but not to engage in phagocytosis, leaving this activity to professional phagocytes.
Class A SRs are homotrimeric type II transmembrane proteins (extracellular C-terminal). SRA exists as two splice variants (SRAI and SRAII) of a single gene whose primary function is to promote the phagocytosis of a range of nonopsonized bacteria, including S. aureus, Streptococcus pneumoniae and Escherichia coli . SRA-deficient mice have increased susceptibility to systemic infections with S. aureus and Listeria monocytogenes and to pulmonary infections with S. pneumoniae. Macrophage receptor with collagenous structure (MARCO) is an additional class A SR that is involved in innate pulmonary host defense against S. pneumoniae. Down-regulation of MARCO by IFN-γ increases susceptibility to S. pneumoniae in mice and may contribute to the development of pulmonary bacterial pneumonias following influenza infections. MARCO may also play a role in dampening pulmonary inflammation because MARCO-deficient mice exhibit increased lung inflammation in response to inhaled silica and oxidants.
Class B SRs are primarily represented in the lung by CD36. CD36 is expressed on monocytes, macrophages, DCs, and vascular endothelium. Like class A SRs, CD36 has been implicated in PAMP recognition and in the phagocytosis of S. aureus. However, whereas CD36 deficiency in mice results in impaired host defense against S. aureus, the absence of CD36 in humans, via a natural genetic deficiency, is not associated with a pulmonary phenotype.
C-Type Lectin Receptors.
C-type lectin receptor (CLR) domains are also present in a family of cell surface receptors that play an important role in the function of DCs and macrophages (see Fig. 12-3C ). CLRs recognize high-density carbohydrate-based PAMPs on microbial cell walls and viral coats. Carbohydrate binding can be divided into mannose and galactose specificity. Type I CLRs include dendritic and thymic epithelial cell-205 (DEC205) and macrophage mannose receptor, and type II CLRs include dendritic cell-specific intracellular adhesion molecule (ICAM3), dendritic cell–specific intercellular adhesion molecule–grabbing nonintegrin (DC-SIGN), and dendritic cell-specific receptor-1 (DECTIN1). As we discuss later, studies in mice bearing targeted disruptions of CLR genes have emphasized their importance in innate host defense and particularly in DC maturation and antigen presentation.
DEC205 is a type 1 transmembrane receptor protein found on a variety of DC subsets and macrophages. The natural carbohydrate ligands remain largely uncharacterized, though the receptor has been shown to induce the endocytosis of experimental antigen–anti-DEC205 receptor complexes and promote their delivery to endosomes before antigen processing and presentation. When DCs are treated with the antigen–anti-CD205 fusion protein alone, they induce tolerance to the antigen by deleting specific CD4 + and CD8 + T cells and by promoting the development of T regulatory (Treg) cells. In the presence of an inflammatory stimulus, DCs exposed to the antigen–anti-DEC205 fusion proteins promote long-lived immunity mediated by Ag-specific CD4 + and CD8 + T cells. Antigens delivered by targeting DEC205 may be presented in association both with MHC class II molecules and by cross-presentation with MHC class I molecules. Furthermore, a recent study showed that DEC205 mediates the uptake of self-antigens via the endocytosis of apoptotic cells, thereby providing a plausible mechanism for the cross-presentation of self-antigens resulting in the induction of both central and peripheral tolerance.
DECTIN1 was initially thought to be expressed specifically on DCs but was later shown to be expressed also on macrophages, monocytes, PMNs, and some T-cell subsets. DECTIN1 specifically recognizes β-(1,3)-linked glucans and β-(1,6)-linked glucans and is thought to play a key role in the nonopsonic phagocytosis of a number of pathogenic yeasts, fungi, and bacteria by macrophages and DCs. Ligation of DECTIN1 also stimulates the production of an array of proinflammatory cytokines, oxidants, and lipid mediators.
DC-SIGN is also a type II CLR but differs from DECTIN1 by its specificity for microbial mannose-rich carbohydrates. DC-SIGN is important in DC trafficking and DC–T-cell interactions through its ability to interact with ICAM3 and ICAM2, respectively. DC-SIGN is also expressed on the surface of a subset of peripheral blood and lung BDCA-2 + plasmacytoid DCs (pDCs) and resident alveolar macrophages. Interestingly, increased expression of DC-SIGN by alveolar macrophages has been reported in response to stimulation with interleukin-13 (IL-13), suggesting a possible role for DC-SIGN + cells in the pathogenesis of type 2 T helper (Th2)–mediated lung diseases.
Cytoplasmic Pattern Recognition Receptors
Nucleotide-Binding and Oligomerization Domain–like Receptors.
In humans, NLRs are a family of 22 intracellular PRRs that have evolved to sense PAMPs in the cytoplasm of most cells. Cytoplasmic PAMPs recognized by NLRs include bacterial peptidoglycans and flagellin from gram-positive and gram-negative bacteria as well as microbial toxins such as Bacillus anthracis lethal factor. Earlier, we briefly introduced DAMPs as a collection of nuclear and cytoplasmic molecules, including high-mobility-group box-1 protein, adenosine triphosphate, nicotinamide adenine dinucleotide (NAD + ), and adenosine, that are released following tissue injury. Recent studies have also shown that, though not technically DAMPs, uric acid and crystalline silica are also sensed by NLRs and, like PAMPs, initiate sterile inflammatory responses. In turn, the recognition of cytoplasmic PAMPs and DAMPs by NLRs activates an array of signal transduction pathways that lead to the production of proinflammatory cytokines. NLRs also induce the assembly of intracellular protein-protein complexes called “inflammasomes,” which stimulate IL-1β production and initiate several different forms of programmed cell death, including apoptosis, necrosis, pyroptosis, and pyronecrosis. NLRs may also play a role in the maturation of immature DCs after exposure to PAMPs or cytokines. The precise mechanisms by which NLRs signal is an area of intense investigation, but studies show that NLRs participate in the formation of three distinct inflammasomes, including the NALP1- and NALP3-containing inflammasomes and interleukin-1β–converting enzyme protease–activating factor–containing inflammasomes. Although the role of NLRs in a variety of human disorders has been characterized, the complete role of these molecules in lung homeostasis and defense is not yet fully understood. More information about NLR structure and function is available in several recent reviews.
Retinoic Acid–Inducible Gene-1–like Receptors.
The last group of cytoplasmic PAMP sensors is the RIG1-like receptors that have evolved to detect the presence of RNA from RNA viruses and replicating DNA viruses. Three family members, RIG1, myeloma-differentiation associated gene 5, and laboratory of genetics and physiology 2, have been identified. RIG1 has been shown to recognize viral RNA sequences from several viruses and is involved in the response of lung epithelial cells to influenza virus. In contrast, myeloma-differentiation associated gene 5 has been shown to respond to polyriboinosinic:polyribocytidylic acid and picornoviruses. Upon sensing viral RNA sequences, RIG-like family members promote the expression of type I IFNs and proinflammatory cytokines, thereby inducing and augmenting host and lung innate immunity.
Summary
Secreted, plasma membrane, endosomal, and cytosolic PRRs provide remarkable flexibility in innate protection of the lung. In the descending airways, plasma membrane PRRs, especially TLRs, are poised to sense PAMPs but are generally unable to phagocytose PAMP-containing microbes. However, TLR signaling in airway epithelial cells results in the production of proinflammatory mediators, especially chemokines and cytokines, which call in PMNs and macrophages to the site of PAMP detection to enable microbial clearance. In addition, intraepithelial DCs constantly sample the airway lumen to maintain immunologic tolerance to commonly encountered antigens that do not express PAMPs, while remaining poised to activate the adaptive immune system once PAMPs are sensed. The alveoli are also protected by epithelial cells, DCs, and resident alveolar macrophages that also sense PAMPs via plasma alveolar PRRs. However, in the alveoli, two additional levels of protection exist. First, secreted PRRs, especially SP-A and SP-D, protect the alveolar surfaces by tonically suppressing unwanted inflammation in the steady state, while remaining poised to stimulate innate responses in the presence of PAMPs. Second, resident alveolar macrophages express an additional array of PRRs, especially SRs that promote the phagocytosis of microbes and other particulates. It seems reasonable to suggest that, in the steady state, the innate immune system responds to inhaled particulates and microbes continuously, but silently. In the next section, we discuss how ligation of these various PRRs activates and regulates innate host defense responses in lung cells.
Effector Mechanisms
The cell types that are primarily responsible for innate protection of the lung in the steady state are (1) airway and alveolar epithelial cells and (2) macrophages and DCs. Additionally, in response to the initial activation of these cells, PMNs, which though not an abundant cell type in the steady state, are rapidly recruited to augment lung phagocyte numbers once the presence of PAMPs is detected. In the following section, we review the origin and functions of these resident and recruited cells in innate protection of the lung.
Epithelium
The lung epithelium has an important role in host defense against microbes that pass through the glottis and reach the conducting airways and gas-exchange parenchyma. The conducting airways of the lower respiratory tract are lined by ciliated columnar epithelial cells down to the terminal airways; these cells become nonciliated in the respiratory bronchioles. In contrast, the alveolar epithelium consists of flattened type I epithelial cells that form most of the alveolar surface and type II cuboidal epithelial cells that produce surfactant and surfactant-related proteins and project into the subepithelial structures of the lungs. The classic antimicrobial defense mechanism in the conducting airways is the mucociliary system, which moves microbes deposited on the airway epithelial surface upward and out of the lungs. In addition to this physical removal system, the airway and alveolar epithelium participate actively in the innate defense of the lungs, with the major goal of protecting the critical gas-exchange surface from microbial invasion. The mucociliary system provides for the removal of all particles that deposit on the airway epithelium, and the clearance times in the trachea and proximal airways are measured in minutes (see ). The cilia on the epithelial surface beat in coordinated waves, directing the movement of particles upward toward the larynx. Epithelial cell activation is not required for optimal ciliary beating, although beat frequency can be speeded by β-agonists and slowed by opiates and other drugs. Additional information on mucociliary clearance can be found in Chapter 11 .
The mucociliary system and antimicrobial constituents of airway epithelial fluid discussed in the “Overview of the Components of Lung Innate Immunity” section (see Table 12-1 ) can be thought of as constitutive host defenses, because they do not depend on specific microbial recognition mechanisms and do not need activation. However, the airway and alveolar epithelium also participate in innate immune mechanisms in that they express bacterial recognition molecules (PRRs) common to innate immune cells and can produce an array of proinflammatory mediators that recruit leukocytes into the airways directly through the airway and alveolar epithelial walls. Bacterial products stimulate the airway epithelium to produce chemotactic signals that recruit inflammatory cells into the airways. Endogenous cytokines also stimulate airway and alveolar epithelial cells to amplify leukocyte migration. Bacterial LPS stimulates ciliated airway epithelial cells to produce CXC and CC chemokines, which recruit PMNs and monocytes, respectively, into the airway lumen. Airway epithelial cells also produce IL-1β, IL-6, IL-8, RANTES (regulated on activation, normal T-cell expressed and secreted), granulocyte-macrophage colony-stimulating factor (GM-CSF), and transforming growth factor-β (TGF-β). As with other cells involved in innate immunity, airway epithelial cells produce cytokines via the activation of transcription factors, including nuclear factor-κB (NFκΒ), activator protein-1, and nuclear factor interleukin 6. Interestingly, noninfectious environmental agents like ozone, asbestos, diesel exhaust particles, and air pollution particles all lead to NFκΒ activation in airway epithelial cells under experimental conditions, which is typically followed by IL-8 production and release. Airway epithelial cells also recognize unmethylated bacterial DNA via TLR9, leading to NFκΒ activation and production of IL-6, IL-8 and β 2 -defensin in the airways. Unlike airway epithelial cells, alveolar epithelial cells do not respond directly to LPS but do produce chemokines in response to the tumor necrosis factor-α (TNF-α) and IL-1β secreted by alveolar macrophages in response to bacteria or their products.
The critical role of the lung epithelium in innate immunity and microbial defense has been supported by studies using transgenic mice. When a dominant negative IκΒ construct was expressed in the distal airway epithelial cells of mice, thereby preventing NFκΒ activation, airway PMN recruitment in response to inhaled LPS was impaired. This finding supports the importance of bacterial recognition by distal airway epithelial cells in vivo and shows that epithelium-derived cytokines produced by the NFκΒ pathway probably are just as important as macrophage-derived cytokines in driving innate inflammatory responses in the airways and alveolar spaces. Hajjar and colleagues created bone marrow chimeras in which either myeloid (leukocytes) or nonmyeloid (epithelial) cells lacked Myd88, a key adapter molecule required for signaling via all TLRs except TLR3. It turned out that nonmyeloid deficiency of Myd8 impaired bacterial clearance more than myeloid deficiency. Specifically, the mice lacking Myd8 (and therefore lacking TLR signaling) in nonmyeloid cells, including the airway and alveolar epithelium, had markedly impaired clearance of P. aeruginosa, whereas mice with or without Myd8 in myeloid cells had normal bacterial clearance. This surprising result further supports the important role of the airway and alveolar epithelium in bacterial recognition and clearance from the lungs, a role that is perhaps equivalent to that of resident and recruited leukocytes.
Innate immune responses in the airway epithelium can generate or influence adaptive responses. For example, recognition of β-(1,3)-glucan in house dust mites stimulates the production of CCL20, a chemokine that recruits immature DCs into the airways. The airway epithelium can directly influence the function of lung DCs, inducing adaptive Th2 responses that are thought to be important in the pathogenesis of asthma, and LPS responses in the airway epithelium are involved in some DC-driven Th2 cell responses. Airway epithelial cells produce thymic stromal lymphopoietin, GM-CSF, IL-1β, IL-25, IL-33, and osteopontin, all of which activate DCs.
An emerging concept is that deliberate stimulation of innate immunity in the airways and air spaces produces broad enhancement of antimicrobial defenses. Exposure of mice to a crude extract of nontypeable Haemophilus influenzae bacteria via aerosol initially stimulated innate immunity and then protected the mice from lethal infection with S. pneumoniae . This was associated with enhanced production of lysozyme, lactoferrin, and defensins in the lungs. Similarly, exposure to this crude bacterial extract protected mice from S. aureus, P. aeruginosa, and the fungus Aspergillus fumigatus. Thus prestimulation of innate immune mechanisms in the airway and perhaps alveolar epithelium enhances the antimicrobial activity and host defense of the lungs against a broad array of bacteria and fungi. The implication is that deliberate low-level stimulation of innate immunity in the lungs could be used as a protective strategy; however, the emerging recognition of the important role of lung epithelial innate immunity in stimulating and regulating adaptive immune mechanisms raises the possibility that such a strategy might have unexpected effects.
Thus innate immune mechanisms in the airways stimulate endogenous defenses by enhancing production of airway defensins and other antimicrobial products, by stimulating PMN and monocyte recruitment into the airways to augment antimicrobial defenses, and by setting the stage for DCs and Th2-mediated adaptive immune responses that could be important in the pathogenesis of allergic lung diseases.
Polymorphonuclear Leukocytes
PMNs serve as the immediate effector arm of the innate immune system. PMNs are produced from progenitor cells in the bone marrow, circulate for a short time in the bloodstream, and migrate to sites of tissue inflammation in response to signals produced by local innate immune mechanisms. Mature PMNs are released from the marrow in response to granulocyte colony–stimulating factor and other stimuli and circulate for up to 6 to 8 hours. The bone marrow releases between 10 9 and 10 10 PMNs each day, and marrow release can increase severalfold in response to acute signals from the lungs and other tissues. PMNs contain several different kinds of cytoplasmic granules, which contain an array of proteins ( Fig. 12-4 ). Small specific granules serve as a source of new membrane and fuse with the leading edge of the cell during migration. Primary (azurophilic) granules contain myeloperoxidase, which accounts for the green color of pus, bacterial permeability–increasing protein, PMN elastase, matrix metalloproteinases, other proteinases, and defensins.

Circulating PMNs are spherical, with a diameter of approximately 8 µm and must deform to make their way through the capillary microcirculation in the lungs and other organs. PMNs bear surface adhesion molecules that recognize carbohydrate and protein moieties expressed on activated endothelial cells. In the systemic circulation, PMNs migrate into tissue through postcapillary venules in a four-step process by (1) weakly adhering to the venular endothelium, (2) rolling along the endothelial surface, (3) arresting on the endothelial surface, and (4) migrating between endothelial junctions into tissue in response to local chemotactic gradients. This systemic paradigm is different in the lungs, where the pulmonary capillaries slow the transit of PMNs because of the small cross-sectional capillary diameter. This produces a reservoir of capillary PMNs that are poised to respond directly to signals from the innate immune system in the air spaces. When bacteria or their products such as gram-negative LPS circulate in the bloodstream, PMNs undergo activation with cytoskeletal rearrangements that cause stiffening, promoting increased entrapment of PMNs in the pulmonary capillaries.
When innate immunity is activated in the lungs, chemotactic factors are produced by resident alveolar macrophages and activated epithelium, which create soluble and tissue-fixed gradients that recruit PMNs into the alveolar spaces. Leukotriene B 4 is a product of arachidonic acid metabolism in alveolar macrophages that produces an immediate and short-lived soluble gradient that recruits PMNs into the air spaces but dissipates within minutes. Release of leukotriene B 4 is closely followed by the release of IL-8, a chemokine that binds to heparin residues on tissue matrix, creating a tissue reservoir that promotes long-lived gradients. IL-8 is the dominant member of a group of CXC chemokines, which also include GRO-α, GRO-β, and GRO-γ, and ENA-78. The CXC chemokines have a C-X-C sequence at the N-terminus, which provides PMN specificity, and an N-terminal Glu-Leu-Arg peptide sequence, which is important in chemotactic receptor binding. The CXC chemokines recruit PMN to sites of inflammation, whereas chemokines with the CC N-terminal sequence recruit monocytes. IL-8 and related chemokines probably reach the endothelial luminal surface by diffusion from alveolar fluids and bind to endothelial cell surface glycosaminoglycans to create a fixed local signal that guides PMNs sequestered in pulmonary capillaries into the air spaces. Whereas PMNs bear a single receptor for leukotriene B 4 , they express two different receptors with differing affinity for IL-8. The low-affinity receptor (CXCR2) is thought to recruit PMNs to sites of inflammation, whereas the high-affinity receptor (CXCR1) is thought to guide PMN migration into the tissues. The low-affinity receptor is shed from the surface of circulating PMNs in sepsis, leaving the high-affinity CXCR1 receptor as the dominant IL-8 receptor. This suggests that a CXCR1 receptor–targeted strategy could be effective in limiting PMN inflammation in patients with sepsis. PMNs also have receptors for the complement component C5a and for formylated peptides produced by bacteria in the air spaces, so that endogenous as well as exogenous signals attract PMNs to sites of inflammation. The diversity of chemotactic stimuli recognized by PMNs promotes the formation of combinatorial gradients that guide PMN migration toward localized sites of inflammation in the lungs and other tissues.
In the systemic circulation, PMN migration into tissues depends on the integrin CD11/CD18 on the PMN surface recognizing the counterligand ICAM1 on the endothelial surface. In the lungs, however, both CD18-dependent and CD18-independent mechanisms exist, and the signals that determine the dependence on CD18 are not clear. For example, PMN migration into the lungs in response to E. coli and P. aeruginosa is CD18 dependent, whereas PMN migration in response to S. pneumoniae does not require CD18. TNF-α and IL-1β appear to direct CD18-dependent migration, whereas IFN-γ is more important for CD18-independent migration.
Under normal circumstances, PMN migration from the blood into the air spaces is not associated with injury to the endothelial or epithelial barriers. At the endothelial barrier, endothelial cells undergo reversible contraction in response to thrombin and other inflammatory stimuli, thereby opening endothelial junctions and allowing the passage of leukocytes. The alveolar barrier is much tighter, and yet PMNs reach the air spaces with only minor and transient changes in epithelial permeability. During migration the spherical PMNs in the bloodstream change shape, and small specific granules fuse with the leading edge of the cell membrane, providing new membrane material bearing specific adhesion molecules. PMNs in the air spaces are polarized, which is an initial sign of activation, but contain the full complement of primary azurophilic granules containing myeloperoxidase and defensins. Metabolic studies of PMNs in rabbits with pneumococcal pneumonia and humans with bronchiectasis have shown that most PMNs become activated in the air spaces and not during migration into the lung. However, when endothelial activation is intense or when PMN activation signals are present within the circulation, PMNs become preactivated and migration can be associated with damage to the endothelial and epithelial barriers, with the development of increased-permeability pulmonary edema.
Once in the air spaces, PMNs ingest bacteria and fungi that have been opsonized by complement and immunoglobulins that accumulate in the air spaces at sites of inflammation. PMNs contain a series of effector mechanisms to kill bacteria and fungi, including oxidant production, microbicidal proteins in primary azurophilic granules, and extracellular traps (see Fig. 12-4 ). As microbes are recognized and the phagosome begins to form, the subunits of a nicotinamide adenine dinucleotide phosphate , reduced form (NADPH) oxidase are assembled in the cell membrane, and the cell undergoes a respiratory burst, in which an electron is transferred to molecular oxygen to form superoxide anion, which is reduced again to form hydrogen peroxide. This happens on the invaginating phagosomal membrane, focusing oxidants on the contents of the developing phagosome. As the phagosome forms, the primary granules fuse with the phagosomal membrane, adding myeloperoxidase and cationic antimicrobial peptides to the phagolysosome. Myeloperoxidase catalyzes the formation of hypochlorous acid from hydrogen peroxide and a halide, typically chloride (Cl − ) because of its high concentration in the cellular environment. Hypochlorous acid is a highly reactive oxidant that oxidizes methionines, tyrosines, and other amino acids on proteins, killing the microbe.
In addition to their presence in airway surface liquid, the primary azurophilic granules also contain high concentrations of the α-defensins, human neutrophil protein 1, 2, and 3. When defensins are added to the phagolysosomal space, they attach to negatively charged microbial membranes via electrostatic interactions and are thought to form lytic pores in the microbial cell wall. PMN defensins have antimicrobial activity for gram-positive and gram-negative organisms, fungi, and some viruses. Defensins are most active under conditions of low ionic strength and lose activity with increasing concentrations of salt or plasma proteins, which interfere with electrostatic interactions between the cationic defensins and the anionic microbial surface. The loss of defensin activity in higher salt concentrations has been proposed as a contributing factor for the pathogenesis of chronic airway infection in cystic fibrosis. In addition to their antimicrobial activity, defensins participate directly in innate and adaptive immunity, because they stimulate IL-8 production by epithelial cells and modulate the responses of T cells and immature DCs.
When phagocytosis is appropriately regulated, microbes are killed within the protected environment of the PMN phagolysosome. However, at sites of intense inflammation, PMNs release superoxide anion, hydrogen peroxide, and granular contents directly into the extracellular environment, leading to oxidant formation in the alveolar spaces with oxidation of structural proteins in the alveolar walls and intracellular proteins in leukocytes, and the accumulation of defensins and other granular contents in the alveolar spaces. These extracellular products contribute to indirect tissue injury by PMNs.
In addition to killing intracellular microbes using oxidants, chlorination, and antimicrobial peptides, as a final act, PMNs can project uncoiled nuclear DNA into the surrounding environment to form neutrophil extracellular traps (NETs) that ensnare and destroy bacteria. NET formation depends on the initial respiratory burst of the PMN and leads to the death of the PMN in a process that is distinct from apoptosis and necrosis. The PMNs of patients with chronic granulomatous disease, which lack a functional membrane NADPH oxidase, do not form NETs following appropriate stimulation. A variety of proinflammatory stimuli activate NET formation, including LPS and IL-8. C5a triggers NET formation in PMNs after priming with IFNs or GM-CSF. Some microbes, including S. aureus, E. coli, P. aeruginosa, and M. tuberculosis, directly stimulate NET formation by PMNs. The extracellular DNA mesh contains cationic proteins embedded in the negatively charged DNA net, including histones, defensins, and cathelicidin, which kill enmeshed bacteria. Similar extracellular traps are produced by eosinophils and mast cells, but unlike PMNs, eosinophils apparently survive NET formation. NET formation thickens secretions at sites of inflammation, creating a viscous pus that contains enmeshed microbes. As might be expected, some bacteria produce extracellular enzymes that degrade DNA NETs, including Streptococcus pyogenes (DNase Sda1/2) and S. pneumoniae (DNase EndA). The mechanisms by which NETs are cleared from the air spaces during resolution of inflammation are not clear.
PMNs have an important role in signaling the activation of adaptive immunity. In rabbits with tuberculous pleurisy, PMNs are the first cells that migrate into the infected pleural space, where they produce chemotactic factors that direct the subsequent wave of monocyte recruitment, which characterizes the full inflammatory reaction to M. tuberculosis. PMN granule proteins cathepsin G and azurocidin are chemoattractants for mononuclear cells, which mature into macrophages and DCs at sites of inflammation, and neutrophil-derived CC chemokines recruit DCs. PMNs release limited amounts of TNF-α and IFN-γ, which regulate macrophage, T-cell, and DC activation and maturation; however, the large numbers of PMNs at sites of inflammation can bring the concentrations of these PMN-derived cytokines into biologically relevant ranges. PMNs also produce CXC chemokine ligand 10 (IFN-γ inducible protein-10), which is a chemoattractant for natural killer cells and type 1 T helper (Th1) cells.
At the same time that PMNs participate in and intensify inflammation in tissue, they also produce signals that control and begin the resolution of inflammation. PMNs, like macrophages and endothelial cells, release the secretory leukocyte protease inhibitor, which inhibits PMN elastase. After migration into tissues, PMNs produce lipoxins from membrane arachidonic acid, which inhibit PMN recruitment, superoxide anion generation, and NFκB activation and enhance the uptake of apoptotic PMNs by macrophages. PMN-derived oxidants inactivate proteases and other proteins at sites of inflammation.
PMNs and their products are cleared largely by macrophages during the resolution of inflammation. PMNs die primarily by apoptosis, necrosis, or NET formation. Apoptosis is a highly regulated form of cell death and is mediated by a series of intracellular caspases. Apoptosis is mediated via two major pathways: ligation of membrane death receptors, principally FAS/CD95 and TNFRI (p55), and mitochondrial stress that results in the release of cytochrome c into the cytoplasm. PMNs undergoing apoptosis express phosphatidylserine on the outer leaflet of the cell membrane and undergo nuclear chromatin condensation and cell shrinkage. Apoptotic PMNs are recognized by macrophages via the class B SR, CD36, and are rapidly ingested, so that large numbers of apoptotic PMNs are usually not seen. Macrophages that ingest apoptotic PMNs produce TGF-β and IL-10, which have anti-inflammatory effects. This process results in the clearance of PMNs and their residual intracellular contents and dampening of inflammation. PMNs that are not ingested by macrophages undergo secondary necrosis with loss of membrane integrity, cytoplasmic swelling, and release of remaining intracellular contents into the inflammatory environment. Intracellular components are recognized as danger signals by macrophages, some of which have been included as “alarmins,” or DAMPs. DAMPs include the nuclear protein high-mobility-group box-1, granular antimicrobial peptides, and other intracellular products. Macrophages that recognize these danger signals produce IL-1β, TNF-α, IL-8, and other proinflammatory cytokines, initiating or perpetuating inflammatory responses. Some PMNs recovered from the lungs of patients with acute respiratory distress syndrome have features of apoptosis, with small cytoplasmic features and nuclear pyknosis, whereas many have features of necrosis, including severe degranulation, membrane blebbing, and cytoplasmic swelling. The signals that govern the balance between necrosis and apoptosis are incompletely understood.
Thus PMNs are important effector cells in innate immunity, ideally designed to circulate through the body and accumulate rapidly at tissue sites of acute inflammation. Under normal circumstances, they arrive in tissue ready to ingest and kill microbes, then quietly go away by programmed cell death. PMN-derived signals regulate local inflammation and stimulate adaptive immune responses, providing a broader role for PMNs in host defense.
Mononuclear Phagocytes
The concept of the mononuclear phagocyte system as a functionally and phenotypically heterogeneous system of mononuclear phagocytes distributed throughout the body was developed in the 1960s and 1970s through a series of insightful studies pioneered by van Furth and Cohn and Volkman and Gowans. The central concept was that the resident macrophages of all organs and tissues were derived from progenitor cells in the bone marrow. In turn, the progenitor cells gave rise to circulating blood monocytes that were recruited into organs and tissues, where they differentiated into macrophages, to maintain macrophage homeostasis. In response to additional needs during inflammation or infection, monocyte production in the bone marrow increases, thereby enabling increased monocyte recruitment to affected tissues. Although many aspects of this conceptual framework have been confirmed, recent studies building on earlier foundational work have challenged the concept that resident macrophages are derived from bone marrow–derived monocytes and have provided important insights into the origins of resident tissue macrophages during early embryonic development. Fate-mapping studies involving lineage-tagged mice and other genetic models have suggested that there is a pool of “primitive” F4/80 bright resident macrophages located within tissues that are derived from the yolk sac beginning on embryonic day 8 (E8) ( Fig. 12-5A ). In addition to expressing high levels of F4/80, these yolk sac–derived macrophages express CX3CR1, macrophage mannose receptor (MMR), and colony-stimulating factor 1 receptor (CSF1R, c-fms ) and are dependent upon IL-34, CSF1, and the transcription factor PU.1 for their development (see Fig. 12-5A ). By E10.5, yolk sac–derived macrophages are found in most tissues, including the lung, and are able to undergo self-renewal. At E10.5 the fetal liver becomes the major site of hematopoiesis, and with its development, a unique “definitive” macrophage population derived from the hematopoietic stem cell can also be found. These cells express low levels of F4/80, high levels of CD11b, and, in contrast to the yolk sac–derived macrophages, are dependent on the transcription factor c-Myb for development. During liver hematopoiesis, macrophages constitute up to 15% of the total cells in many organs, and their importance in embryonic development has been definitively shown in studies using c-fms- , colony-stimulating factor 1–, CX3CR1-, c-Myb– and PU.1-deficient mice. These studies also showed that adult Langerhans cells, the prominent antigen-presenting cells of the skin, are produced by the fetal liver and that their development is dependent on IL-34 and CSF1R. In studies using these and other models, macrophages have been shown to play important roles in bone morphogenesis, ductal branching in the mammary gland, neuronal patterning, angiogenesis, vascular remodeling, and in kidney and endocrine development. Analysis of embryonic tissue for yolk sac–derived and hematopoietically derived macrophage populations has shown that the lungs basally contain macrophages of both yolk sac and hematopoietic stem cell origin. However, a more detailed analysis of the precise location of these subpopulations (e.g., airway or interstitium), their unique cell surface marker profiles, and how they interact with other pulmonary cells during development has yet to be conducted. Macrophages may contribute to lung development through the clearance of cellular debris associated with tissue remodeling and through the production of growth factors that influence the airway epithelium. Recently, colony-stimulating factor 1 receptor–positive macrophages with a “remodeling” phenotype have been shown to be located near branch points in the lung during branching morphogenesis, though the origin and location of these cells within the adult lung remains unknown.

In addition to macrophages, DCs play a fundamental role in lung innate immunity. Steinman published the first report on a population of antigen-presenting cells in mouse spleen and, based on their morphologic characteristics, coined the name dendritic cells ( DCs ). This work was recognized in 2012 with the posthumous award of the Nobel Prize in Physiology or Medicine. Since the late 1980s a vast array of monoclonal antibodies against monocytes, macrophages, and DC cell surface antigens, together with lineage-tracing mice, have been developed and used to classify monocytes/macrophages and DCs. The issue of using cell surface markers to separate macrophages and DCs is particularly relevant in the lung, where CD11c is expressed at high levels on resident alveolar macrophages, and DCs (as reviewed by Hume ). In addition, much of our initial understanding of the functions of resident lung mononuclear phagocytes has come from studies conducted without the use of extensive cell surface marker panels or lineage-tracing technologies. Thus, although it is clear that the cells described in many studies are mononuclear phagocytes, it is sometimes difficult to classify them further without the use of multiple antibody markers combinations. As illustrated in Figure 12-5B , pulmonary mononuclear phagocytes can be broadly thought of as three overlapping subpopulations: (1) resident macrophages of the alveolar surfaces and interstitium, (2) monocytes that marginate in the lung microvasculature and can be recruited into the air spaces in response to innate activation, and (3) resident and recruited DCs of the airways and lung parenchyma. In this section, we discuss the localization and innate immune functions of each group of cells.
Resident Macrophages
Resident alveolar macrophages reside in the mixed environment of epithelial lining fluid and ambient inhaled air, whereas resident interstitial macrophages are located within the interstitial tissue or alveolar septa. Morphometric analyses indicate that the number of interstitial macrophages in normal lung is between one tenth and one half of the total number of alveolar macrophages. Unlike resident alveolar macrophages that can be obtained by bronchoalveolar lavage, interstitial macrophages can be obtained only by using tissue dispersion techniques. Consequently, considerably less is known about the biology and function of interstitial macrophages than about resident alveolar macrophages, especially in humans. In addition, interpreting current and past studies can be difficult because artifacts in cell phenotype and function can be introduced as a consequence of the tissue dispersion approach or by in vitro exposure to trace amounts of contaminating PAMPs during isolation. Despite these caveats, interstitial macrophages are thought to share many functional similarities with resident alveolar macrophages.
Functions of Resident and Interstitial Alveolar Macrophages.
The primary functions of resident alveolar macrophages are (1) to dispose of inhaled microbes and particulates, (2) to clear pulmonary surfactant, and (3) to suppress the development of inappropriate inflammatory and immune responses. Resident alveolar macrophages are capable of phagocytosing a wide spectrum of harmless and harmful microbes and other particulates. Under basal conditions, most ingested phagocytosed particulates are enclosed within phagosomes, which ultimately fuse with lysosomes, leading to their degradation by an array of acid-pH optimum hydrolytic enzymes. Some inhaled microbes (e.g., M. tuberculosis ) and some environmental particulates (e.g., crystalline silica) are resistant to this process and become sequestered in secondary lysosomes where they remain for the life span of the macrophage. Most of these latter cells probably crawl into the airways and are cleared via the mucociliary escalator. Some particle-laden macrophages may remain in the lung for extended periods of time before either dying and releasing their particle burden, thereby rendering it available for phagocytosis by other macrophages, or undergoing apoptosis and being cleared by other phagocytes.
Resident alveolar macrophages also actively contribute to the normal homeostasis of pulmonary surfactant. This point is most clearly emphasized in patients with pulmonary alveolar proteinosis, a disorder characterized by the accumulation of proteinaceous and lipid-rich surfactant in the alveoli, leading to impaired gas exchange. A fundamental characteristic of the disorder is the finding that alveolar macrophage numbers are reduced, and those that are present are inefficient in clearing pulmonary surfactant. Several studies have shown that pulmonary alveolar proteinosis is associated with the development of autoantibodies against GM-CSF, emphasizing the importance of GM-CSF in the activities of resident alveolar macrophages. Additional information about alveolar proteinosis can be found in Chapter 70 .
Lastly, resident alveolar macrophages play a critically important role in tonic suppression of alveolar inflammation and adaptive immunity. Based in part on studies by Maclean and associates, the concept has evolved that the lung can deal with inhaled microbes and particulates until a certain threshold burden is reached. The threshold is in part determined by the phagocytic capacity of alveolar macrophages. Once the threshold is exceeded, microbes and other particulates are phagocytosed by resident DCs that “snorkel” through tight junctions of the alveolar epithelium to sample the alveolar compartment. Consistent with this concept, studies have shown that depletion of alveolar macrophages with liposome-encapsulated clodronate augments antigen presentation by pulmonary DCs, which in turn augments adaptive immune responses to intratracheal antigens. Other studies have shown that resident alveolar macrophages suppress the functions of natural killer cells and plasma cells.
The ability of resident alveolar macrophages to suppress inflammation and T-cell responses is specific to these cells and is not a characteristic of macrophages obtained from other locations or peripheral blood. These findings suggest that the alveolar microenvironment may play an important role in the development of suppressive activity by resident alveolar macrophages. As noted earlier, the pulmonary collectins SP-A and SP-D inhibit PAMP-dependent production of proinflammatory cytokines through their interaction with signal-inhibitory regulatory protein α. In addition, TGF-β, produced by alveolar macrophages and activated at the surface of alveolar epithelial cells by the integrin αvβ6, has been shown to basally suppress alveolar inflammation. Other studies have emphasized the importance of IL-10, prostaglandin E 2 , and nitric oxide in the tonic suppression of alveolar inflammation and adaptive immunity. Together these studies suggest that resident alveolar macrophages have several mechanisms that actively suppress alveolar inflammation and the antigen-presenting activity of interstitial DCs, while remaining poised to reverse this response upon appropriate stimulation.
The tonic suppressive activity of alveolar macrophages can be reversed in two different ways. One mechanism involves exceeding the threshold phagocytic capacity of resident alveolar macrophages, thereby allowing microbes to interact with other lung cells, for example, pulmonary DCs or epithelial cells, which respond to PAMPs and other molecules by producing proinflammatory cytokines. Indeed, GM-CSF and TNF-α have been shown to reverse tonic suppression of adaptive immunity in vivo. A second mechanism involves alveolar macrophages themselves. Here, PAMP recognition by the globular head groups of SP-A and SP-D enables lung collectins to interact with macrophages via calreticulin and CD91. In contrast to the suppressive signal initiated when SP-A and SP-D interact with macrophages via signal-inhibitory regulatory protein α in the absence of PAMPs, CD91 signaling leads to the production of proinflammatory cytokines, which then augment inflammation and the recruitment of PMNs and monocytes. Thus resident alveolar macrophages are capable of suppressing inappropriate alveolar inflammation and adaptive immune responses to commonly encountered antigens. However, mechanisms exist to overcome this suppression to allow the recruitment of inflammatory cells, especially PMNs and monocytes, and to promote adaptive immunity.
Recruitment of Mononuclear Phagocytes
After birth and postnatal bone formation, liver hematopoiesis declines and is replaced by bone marrow hematopoiesis, which then becomes the exclusive source of circulating monocytes. Circulating monocytes have the potential to differentiate into macrophages or DCs, and until recently it was thought that circulating monocytes contribute to the replenishment of resident lung macrophages. However, recent incisive studies have clarified the extent and circumstances under which monocytes replenish resident macrophage and DC populations. In 1989 Passlick and associates reported heterogeneity among human macrophages based on the differential expression of CD14 and CD16. “Classic” CD14 hi CD16 − monocytes make up approximately 95% of circulating monocytes in the steady state, and “nonclassic” CD14 lo CD16 + monocytes constitute the remaining 5%. Similar to human monocytes, mouse monocytes have also been classified into classic (Ly6C hi (GR1 + )CCR2 + CX 3 CR1 lo ) and nonclassic (Ly6C lo (GR1 lo )CCR2 lo CX 3 CR1 hi ) monocyte subsets. During steady-state conditions Ly6C lo monocytes circulate through tissues and patrol the lung microvasculature (see Fig. 12-5A ). This subset also expresses high levels of fractalkine receptor (Cx 3 CR1), which interacts with fractalkine (CX 3 CR1L) expressed on the luminal face of vascular endothelial cells and promotes heterotypic adherence. The Ly6C hi monocyte population transmigrates into tissue under inflammatory and injury conditions in a CCR2-CCL2 (macrophage chemotactic protein 1)–dependent manner and differentiates into inflammatory macrophages and dendritic cells (see Fig. 12-5A ). Ly6C hi monocytes have also been recognized to be a short-lived obligatory precursor intermediate for Ly6C lo monocytes under steady-state conditions. Jakubzick and colleagues recently provided additional insights by showing that, under steady-state conditions, circulating Ly6C hi monocytes can also constitutively traffic into the lung and lymph nodes without differentiating into resident macrophages or dendritic cells. Rather, these Ly6C hi monocytes acquire antigen and traffic to regional lymph nodes (see Fig. 12-5A ). Additional information about the mechanisms of monocyte, macrophage, and dendritic cell migration can be found in Bromley and associates and Springer and colleagues.
Functions of Recruited Monocytes and Macrophages.
In contrast to resident alveolar macrophages, recruited monocytes and macrophages have important proinflammatory and host-defense activities including (1) microbial killing and (2) amplification of inflammation. Monocytes and macrophages kill microbes with reactive oxygen species and reactive nitrogen species. Superoxide anion ( ) is mainly produced by the phagocyte NADPH oxidase. Like PMNs, monocytes subsequently convert
into additional reactive oxygen species in a myeloperoxidase-dependent fashion, but as they differentiate into macrophages, intracellular myeloperoxidase content declines and additional reactive oxygen species (e.g., hydroxyl radical [OH − ] are formed by the Fenton reaction.
The generation of reactive oxygen species is critical to host defense against commonly encountered and pathogenic bacteria. Patients with chronic granulomatous disease and mice bearing a targeted disruption of the p47 phox component of the NADPH oxidase are deficient in their ability to control pulmonary and other infections. However, inappropriate production of reactive oxygen species by macrophages (and other inflammatory cells) can result in epithelial injury that can lead to fibroproliferation, as can be seen with survivors of acute respiratory distress syndrome and patients with idiopathic pulmonary fibrosis, asbestosis, or silicosis. Thus, whereas reactive oxygen species play a vital role in the protection of the lung against microbes, inappropriate production in response to nonmicrobial particulates and pollutants, including cigarette smoke, can result in a spectrum of injury to the airway and alveolar epithelium. Nitric oxide (NO) produced by inducible nitric oxide synthase (iNOS or NOS2) also contributes to macrophage-mediated microbial killing and epithelial injury following condensation with to form peroxynitrite. Whereas the importance of NO in microbial killing of L. monocytogenes and M. tuberculosis has been clearly demonstrated in mice, the role of NO in host defense in humans is less clear.
Recruited monocytes and macrophages also amplify inflammation through the production of cytokines, chemokines, and lipid mediators. Studies conducted in the late 1970s indicated that macrophages hydrolyze arachidonyl-containing phospholipids in response to exposure to a variety of TLR agonists through the actions of cellular and secreted phospholipase A 2 enzymes. The hydrolysis of arachidonyl-containing phospholipids by phospholipase A 2 enzymes results in the formation of (1) lysophosphatidylcholine, which, upon acetylation, leads to the production of platelet-activating factor and (2) arachidonic acid, which serves as a substrate for the production of all eicosanoids (as reviewed by Riches and coworkers).
Platelet-activating factor is produced within minutes of exposure of macrophages to TLR agonists and has broad proinflammatory activities that promote PMN recruitment, bronchoconstriction, and vasodilation. Platelet-activating factor also primes PMNs, monocytes, and macrophages for enhanced production of proinflammatory cytokines in response to PAMPs. Arachidonic acid is oxidized to prostaglandin E 2 , prostaglandin D 2 , prostaglandin I 2 , and thromboxane A 2 by cyclooxygenase-1 and cyclooxygenase-2, and these prostaglandins have important roles in the regulation of vascular tone and in the feedback inhibition of macrophage effector functions. The principal leukotriene produced by macrophages is leukotriene B 4 , which is synthesized following TLR engagement and represents the major immediate PMN chemotactic factor produced in the lung before chemokine expression.
Whereas the production of lipid mediators contributes to aspects of early lung inflammation, the production of cytokines and chemokines by macrophages and epithelial cells is necessary for the optimal recruitment of inflammatory cells and for their activation. As discussed earlier, the human chemokine family consists of four closely related subfamilies that have been classified on the basis of the presence and pattern of conserved cysteine residues and have been designated C, CC, CXC, and CXXXC families (as reviewed by Kunkel and coworkers and Keane and Strieter ). The CXC and CC families are critical to the development of pulmonary inflammation. Members of the CXC family containing the Glu-Leu-Arg motif stimulate PMN chemotaxis, whereas family members that lack the Glu-Leu-Arg motif (e.g., CXC chemokine ligand 10) are produced in response to IFN-γ and help regulate angiogenic responses. In contrast, CC family members are involved primarily in the recruitment and activation of mononuclear cells. Macrophages are capable of producing CXC chemokines, especially IL-8, in the settings of acute and chronic lung inflammation in patients with bacterial pneumonia, acute respiratory distress syndrome, idiopathic pulmonary fibrosis, bronchiolitis obliterans with organizing pneumonia, and cystic fibrosis. In addition, CC chemokines, such as macrophage inflammatory protein 1α, are produced by macrophages in interstitial lung diseases.
Recruited monocytes that develop into macrophages are also capable of further differentiation. Based on earlier work in which different PAMPs were found to induce distinct patterns of macrophage gene expression, the concept evolved that different patterns of gene expression could be induced in response to the conditions or stimuli that prevail at the sites to which macrophages have been recruited. For example, exposure of macrophages to Th1 cytokines and TLR ligands (e.g., LPS and polyriboinosinic:polyribocytidylic acid) results in increased expression of nitric oxide synthase 2 (NOS2), TNF-α, IL-12, IL-23, and IL1-β, whereas IL-10 and arginase I expression are repressed. This response generally leads to a “classically activated macrophage” ( Fig. 12-6A ). In contrast, exposure to Th2 cytokines, including IL-4 and IL-13, leads to a different pattern of gene expression characterized by increased expression of arginase I, FIZZ (found in inflammatory zone), and CC chemokine ligand 19 together with reduced expression of NOS2 in an overall response that leads to an “alternatively activated macrophage” (see Fig. 12-6A ). The spectrum of cytokines identified as being capable of inducing alternative macrophage programming is growing and now includes IL-33, IL-21, IL-10, colony-stimulation factors, CXC chemokine ligand 2, CXCL4, glucocorticoids, and TGF-β. In addition, a so-called restorative macrophage programming state that is distinct from classically and alternatively programmed macrophages has recently been identified in self-resolving liver fibrosis (see Fig. 12-6A ).
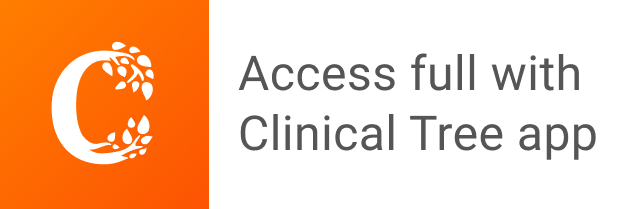