Epilepsy is a chronic neurological disorder that affects approximately 1% of the world’s population, including approximately 3 million people in the United States and 60 million worldwide. Despite the significant advances of pharmacological treatment in the last several decades, 30% to 40% of patients continue to have seizures that are not adequately controlled by antiepileptic drugs (AEDs) (1). Epilepsy surgery has remained the only treatment that can achieve seizure freedom for pharmacologically refractory epilepsy patients (2,3). Scalp electroencephalography (EEG) is an essential component of epilepsy presurgical evaluation for localizing the epileptic focus. When congruent with other noninvasive data, such as ictal semiology and anatomic and functional neuroimaging studies, scalp EEG data can sufficiently guide the surgical resection of an epileptic focus (4–9), particularly in patients with unilateral mesial temporal lobe sclerosis (MTS) and concordant interictal and ictal recordings (10).
However, scalp EEG has intrinsic limitations in its spatiotemporal resolution when attempting an inverse solution (11–13), that is, localizing the cortical source of a given potential. Cortical interictal spikes are typically heterogeneous in their source location, area, synchrony, and amplitude. Only a few of those cortical spikes are associated with scalp-recognizable potentials. Simultaneous scalp and intracranial EEG recording has shown that the cortical area required to generate scalp epileptiform discharges is considerably larger than commonly thought, namely 10 to 20 cm2 (14). Both sufficient cortical source area and synchrony are necessary for the spatiotemporal summation of voltage to produce a scalp EEG epileptiform potential. Additionally, different cerebral source configurations can generate a similar distribution of scalp voltage or magnetic fields. Thus, maximal activity at a certain scalp electrode or sensor does not necessarily indicate that the generators are located in the area underlying it (4,15). Moreover, because the skull attenuates fast frequencies more than slow frequencies, high-frequency and low-voltage rhythms (such as gamma) at ictal onset simply are not recognizable with conventional scalp EEG. Accordingly, scalp ictal rhythms typically reflect propagation and recruitment, rather than indicate the location of seizure-onset zone. False localization and even lateralization are not uncommon, such as in patients with temporal lobe epilepsy (TLE) associated with early contralateral propagation, temporal-plus epilepsies, and extratemporal epilepsy (16–18). Given these intrinsic limitations, scalp ictal recording is often used simply as a screening tool to select those requiring intracranial recordings or to exclude certain patients from further surgical evaluation.
More than a half-century ago, Penfield and colleagues found that seizures in epileptic patients could be cured by removing the brain region from which localized abnormal discharges on EEG (19–22) originated. Since then, identifying the seizure-onset zone on intracranial EEG has become the cornerstone or “gold standard” for localizing epileptic foci. Subdural and/or depth electrodes are commonly used in this task of precisely localizing the epileptogenic focus (23). Additionally, subdural electrodes are well-suited for direct electrical stimulation of the cortex to define the functional (i.e., sensory, motor, and language) areas. Thus, subdural electrodes are useful in achieving both objectives of intracranial EEG study, namely localizing the epileptogenic zone and delineating important functional cortex. This information can subsequently guide a tailored, maximal resection of epileptogenic tissue, while sparing “eloquent” cortex.
HISTORICAL PERSPECTIVES
The success of epilepsy surgery is related to a number of scientific and technological discoveries. In 1870, Gustav Fritsch and Eduard Hitzig found that electrical stimulation of different areas on the cerebral cortex could produce specific motor responses (24). This observation led Hughlings Jackson to believe that focal epilepsy is a manifestation of cortical irritation. He subsequently described Jacksonian seizures (or march) in the primary motor cortex (25,26), and further suggested treatment by removal of the irritating focus. In 1886, Jackson collaborated with neurosurgeon Victor Horsley who performed the first successful epilepsy surgery on a 22-year-old patient with traumatic brain injury and focal motor seizure (27). Horsley subsequently reported 10 similar cases in the following year with mostly favorable outcomes, namely, seizure freedom in two patients and seizure reduction in five patients (28).
This auspicious beginning of epilepsy surgery led to a surge of similar cortical resections in the following decade. However, epileptogenic focus localization by seizure semiology (i.e., motor symptoms) and anatomical identification of the motor strip were notoriously unreliable. Epilepsy surgery was largely saddled with poor outcomes and high morbidity and mortality (29). When phenobarbital was serendipitously discovered as an effective anticonvulsant in 1912, epilepsy surgery suddenly lost its appeal and became largely forgotten.
In 1929, Berger (30) reported the first human EEG recording, which featured the alpha rhythm and alpha block response. EEG in the diagnosis and treatment of epilepsy evolved quickly after Berger’s work. Its usefulness was later confirmed by Adrian and Matthews (31) at Cambridge University in 1934, and by Jasper and Carmichael (32) at Brown University in 1935. In 1935, Foerster and Altenburger (33) performed the first intraoperative electrocorticography (ECoG) during epilepsy surgery. This ECoG technique was soon adopted by Gibbs and Lennox (34–36). Later, Penfield and Jasper (37) used wires and “strip” electrodes during ECoG to localize epileptic focus and determine the extent of surgical resection.
However, intraoperative ECoG is mainly limited to recording interictal discharges, and it is often compromised by anesthetic agents. In order to overcome these disadvantages, chronic intracranial EEG with depth electrodes was first employed in the late 1940s to record spontaneous seizures. Depth electrodes showed to be particularly suited to localize seizure onset in patients with mesial TLE (38–40). Depth electrode recording was further popularized by Bancaud and Talairach with the development of stereotactic technique, which can precisely target subcortical structures (41, 42). This method became known as stereoelectroencephalography (SEEG).
In the meantime, subdural electrodes embedded in a flexible silastic membrane were also gaining in popularity due to a more extensive coverage of cortical surface and easier placement without stereotactic equipment (43, 44). In conjunction with electrical cortical stimulation for functional mapping of the motor, sensory, and language cortex, intracranial EEG with subdural and/or depth electrodes has become both an effective and safe procedure for presurgical epilepsy evaluations.
TECHNICAL CONSIDERATIONS
Subdural versus Depth Electrodes
Several different types of electrodes can be used to record intracranial EEG. These include subdural, depth, and epidural electrodes. Each type has its advantages and limitations. Subdural electrodes are placed directly onto the brain in free-hand fashion to sample epileptic discharges from the cortical surface. They are commonly used to localize epileptogenic foci in the cortical convexity, basal cortex, and interhemispheric cortex. In addition, subdural electrodes, when implanted in large arrays, can determine the extent of an epileptogenic zone for guiding a tailored surgical resection. Moreover, subdural electrodes are well-adapted for cortical mapping, that is, electrical stimulation to localize sensory, motor, and/or language cortex. However, both the implantation and removal of subdural electrodes require major surgery, and, particularly with grids, a large craniotomy. Also, these electrodes cannot localize seizure generators in deep-brain structures.
On the contrary, depth electrodes are commonly used to identify deep epileptogenic foci, such as in the amygdala, hippocampus, insula, and sulcal cortex. They can be also used to determine epileptiform activity in and around hypothalamic hamartoma and periventricular heterotopia. Depth electrodes are commonly placed with stereotactic and/or neuronavigation guidance through small burr holes. These electrodes can be easily removed at the bedside without significant discomfort. Although implantation of depth electrodes is technically more challenging, the procedure is better tolerated by patients and it has lower risks for morbidity. However, depth electrodes provide limited sampling of neocortex compared to subdural electrodes, and they are less good in determining the extent and margins of an epileptogenic focus, particularly if it is necortical (45). In addition, they are not well-suited for functional mapping of the cortex.
In practice, the type of electrode selected for intracranial study is dependent on the strengths and limitations of each type of electrode as determined by the putative location of epileptic focus from noninvasive studies. Many epilepsy centers tend to have a preference regarding subdural versus depth electrodes. For instance, subdural electrodes are commonly used in epilepsy centers in North America, whereas depth electrodes are commonly used in epilepsy centers in Europe. Combinations of subdural and depth electrodes are sometimes used to optimize the yield of an intracranial EEG study; however, this may also increase the accumulative risks of morbidity.
Characteristics of Subdural Electrodes
Subdural electrodes are typically made of stainless steel or platinum-iridium in the form of strips and grids. Subdural electrode arrays come in shapes and sizes that can be customized to the needs of individual patients (Fig. 13.1). Strip electrodes consist of one to two rows of electrodes ranging from four to eight contacts, whereas subdural grids typically consist of four to eight rows of electrodes, typically ranging from four to eight contacts. The contacts are discs mounted on a thin, flexible silastic sheet with 2- to 5-mm diameter exposed to the cortical surface. Intercontact separation is usually 5 to 10 mm. Strip electrodes can be inserted through burr holes. However, a large grid, such as 4 × 4 or 8 × 8 arrays, can only be placed through a sizeable craniotomy, which may increase morbidity, especially with a bilateral grid placement.
Figure 13.1: Commonly used subdural electrodes: (A) 1 × 8 strip; (B) 2 × 8 strip; (C) 4 × 8 grid; (D) 8 × 8 grid. The diameter of each electrode contact is 4 mm. The contacts are spaced at 1.0 cm, center to center. Smaller center-to-center measurements are available. As named, these electrodes are typically placed subdurally or epidurally through a burr hole, if a strip, or through a craniotomy.
The number of subdural electrodes used for a given patient is dependent on the character of the putative seizure focus that was determined in the noninvasive presurgical evaluation by seizure seimology, scalp EEG recording, magnetoelectroencephalography (MEG), and anatomic and functional neuroimaging studies. In one review and meta-analysis, the mean number of implanted subdural electrodes varied from 52 to 95 electrodes, while the mean duration of monitoring may vary from 5 to 17 days, respectively (46). A larger number of electrodes, 100 to 200, can be placed using combination of strip and grid electrodes, particularly when cortical coverage of multiple lobes is clinically indicated. Such a large number of electrodes can exert a mass effect on the brain and cause a midline shift of several millimeters, obviously increasing the risk of surgical complications.
Stainless steel and platinum (usually platinum-iridium) are the typical substrates for subdural electrode contacts. Platinum electrodes have advantages over stainless steel electrodes in that they are nonferrous and thus compatible with magnetic resonance imaging (MRI) and MEG studies. In addition, the denser platinum electrodes have higher signal intensity on head CT images. This can be an important consideration for three-dimensional (3D) CT/MRI coregistration of implanted subdural electrodes, as described in the following section.
Localization of Intracranial Electrodes
From both research and clinical perspectives, the accurate localization of implanted subdural electrodes is critical for delineating the seizure-onset zone and eloquent cortex for planning a tailored resection. With the advent of modern neuroimaging techniques, such as high-resolution MRI, single-photon emission computed tomography (SPECT), positron emission tomography (PET), MEG, and functional MRI (fMRI), the integration of these multimodal diagnostic data has shown great promise in guiding the epileptic focus resection and in improving surgical outcomes (47–49). Key to this multimodal approach is knowing precisely where subdural electrodes, which record epileptiform activity, are located relative to structural and functional imaging results.
Traditionally, localization of intracranial electrodes involved estimating their cortical position based on direct visual inspection through craniotomy, sketches of the basic geometry of the implantation components, and lateral skull x-rays. In the former instance, only the electrodes visible in the open craniotomy can be located. Electrodes placed via burr holes or those positioned over hidden cortical areas, such as temporal tip and basal cortex, cannot be localized reliably. Several advanced quantitative techniques have been developed to locate all implanted intracranial electrodes. These include postimplant 3D MRI images (50), curvilinear reformatting of 3D MRI (51), intraoperative digital photography (52,53), surface reconstruction of CT Scan (54), 3D CT/MRI coregistration (53,55–58), and coregistration of pre- and postimplant MR images (59). While there are advantages and limitations to each method, the 3D CT/MRI coregistration technique is one of the most commonly used. It has the advantage of a realistic display of implanted electrodes relative to cortical anatomy without having electrode artifacts (55). Briefly, both preimplant brain MRI images with volumetric sequences and postimplant head CT images (0.5- to 1.0-mm thin slicing) are obtained. Corresponding pairs of external fiducial anatomic markers (nasion, inion, and preauricular points) from both MRI and CT data sets are identified, and the two volumetric data sets are coregistered to produce an image that shows the electrodes on a 3D cortex (Fig. 13.2).
Figure 13.2: 3D visualization of subdural electrodes obtained from coregistration of a presurgical MRI and postimplant CT in a patient with left TLE. Note the thorough electrode coverage of the anterior and posterior temporal cortex. Combinations of subdural strip and grid electrodes were employed in this study.
Subdural electrode localization via 3D CT/MRI coregistration can be very accurate, but it is dependent on having reliable surface fiducial markers. In a clinical study, this technique was compared to intraoperative digital photography, using gyral/sucal patterns and cortical vasculature as anatomic markers. Localization error was only 2 to 4 mm or approximately half the diameter of the electrode contacts (55,58). Clinically, this is generally acceptable for guiding a tailored resection. In practice, however, the location of surface fiducial markers can be compromised by poor image quality (e.g., motion artifacts) and/or a restrictive field view that precludes identifying scalp landmarks. In such instances, the location error can be significantly increased to 5 to 10 mm, which may pose an unacceptable risk in planning a tailored resection. Therefore, confirmation of electrode localization accuracy when using any coregistration technique should be done with intraoperative digital photography prior to resection (Fig. 13.3).
Figure 13.3: Validation of location accuracy of coregistered subdural electrodes using intraoperative digital photography: A: 3D view of subdural electrodes coregistered onto the patient’s cortex. B: Intraoperative photograph of gyral/sulcal patterns and cortical vasculature visible in the craniotomy prior to electrode placement. Arrow indicates the Sylvian fissure. C: Zoomed-in view of coregistered electrode positions similar to the intraoperative photo. D: Intraoperative view of subdural electrodes in place. The diameter of subdural electrodes (4 mm) and the interelectrode distance (10 mm) were used for the measurement of coregistration accuracy. For example, electrodes 6, 14, 22, and 30 are located on the left superior temporal gyrus. Electrodes 15 and 23 are located on the left frontal operculum. Estimated margin of error was approximately 3 mm.
Risks and Complications of Subdural Electrode Placement
Improvements in grid technology, surgical technique, and postoperative care have resulted in significant reductions in complications following subdural electrode implantation. Morbidity reported in the literature is generally low, however, variable, ranging from 1% to 23% (60–63). Isolated cases of permanent neurological deficits (motor and language) and death have also been reported (61,64–66).
In a recent systematic review and meta-analysis of 21 studies, including a total of 2,542 patients, by Arya and colleagues (46), the most common complications were neurological infection (2.3%), skin/scalp infection (3.0%,), intracranial hemorrhage (4.0%), and elevated intracranial pressure (2.4%). Five deaths (0.2%) were reported as a direct consequence of the subdural EEG study due to refractory elevated intracranial pressure (ICP). Other less-common adverse events included transient motor and language deficits, cerebrospinal fluid leakage, and pneumoencephaly. Electrode removal may be necessary to manage these adverse events, which also results in the premature termination of the intracranial recording. Mild to moderate headache and nausea are also common postimplantation, presumably secondary to increased ICP (67). Management of this condition may require analgesics, fluid restriction, and sometimes corticosteroids. In rare instances, subdural electrodes have also been pulled out by patients (66,68). The risk of complications commonly increases with subdural electrode number (>100), longer duration of intracranial monitoring (>10 days), left-sided implantation, and elderly patients (62,64). Experience is also an important factor. For example, the Cleveland Clinic group reported a 33% rate for minor and major complications at the beginning of their epilepsy program, which dropped to 19% in more recent years (64).
Given that subdural electrode placement can be associated with morbidity and mortality, careful presurgical planning and postimplant patient care is necessary to minimize these. To prevent infection, it is useful to limit the recording period to less than 10 to 14 days. Intraoperatively, the wires of subdural electrodes should be tunneled subcutaneously and brought out at a separate site, where tissues can be tightly sutured. This helps to prevent bacteria from gaining access to the brain and meninges via the exiting wires. In addition, prophylactic use of perioperative antibiotics is a common practice among epilepsy centers.
Subdural hematomas are mainly caused by tearing cortical bridging veins during the insertion of strip and grid electrodes. Such bleeding can be reduced by assuring that coagulation parameters are appropriate prior to surgery. Cerebral edema can also be a serious complication of invasive EEG recording. It may interfere with accurate epileptic focus and eloquent cortex localization. Moreover, cerebral edema that is refractory to intervention can provoke cerebral herniation and result in death. Administration of dexamethasone following surgery and during intracranial monitoring can be useful in reducing cerebral edema; however, it may also decrease the frequency of habitual seizures and thus prolong the duration of intracranial monitoring (69). Therefore, its use needs to be judiciously considered. In some centers, dexamethasone is only administered during the first 72 postoperative hours in patients experiencing significant headache, nausea, or vomiting.
CLINICAL INDICATIONS OF SUBDURAL ELECTRODE EEG RECORDING
Currently, there are no universally recognized guidelines for when an intracranial EEG study is indicated. In practice, the following are common clinical indications for invasive EEG studies (70,71): (a) lateralize ictal onset to a specific hemisphere, such as left versus right temporal epilepsy; (b) localize ictal onset to a specific lobe, such as temporal versus frontal lobe epilepsy; (c) localize seizure onset and determine the extent of seizure-onset zone within a lobe, such as mesial versus neocortical or anterior versus posterior TLE; and (d) functional cortical mapping, such as language mapping in patients with dominant hemisphere TLE. The latter is particularly useful when intraoperative functional mapping is not feasible.
On the other hand, with the advent of modern neuroimaging studies, many epilepsy patients who have unilateral mesial temporal sclerosis or well-defined structural lesions (i.e., glioma and cavernoma), which are concordant with scalp interictal and ictal EEG localization, are usually eligible for direct surgery without the need for an intracranial EEG study (10). Also, subdural studies are not indicated in patients with evidence of multifocal or generalized seizure onsets, since they are not considered good candidates for epilepsy surgery.
POSTOPERATIVE CARE AND EEG ACQUISITION
Postoperative Care
Subdural electrodes are implanted under general anesthesia in the operating room. Afterward, patients are commonly admitted to a neurointensive care unit (NICU) for a brief period of postoperative observation. A head CT and/or brain MRI are usually obtained to rule out acute intracranial hemorrhage and cerebral edema. These neuroimaging studies are also used to localize the implanted subdural electrodes, such as via 3D CT/MRI co-registration (55). Although the choice of antibiotics and duration prophylaxis vary among different centers, antibiotics are commonly administered throughout the course of the intracranial study. Dexamethasone can be also used for patients with signs of increased ICP, such as significant headache and vomiting. Preoperative AEDs are commonly maintained during NICU care or at least until EEG monitoring is begun.
Patients are usually transferred from the NICU to the epilepsy monitoring unit (EMU) within 24 hours to begin EEG recording. AEDs are then typically weaned off gradually to facilitate the recording of habitual seizures. EEG recordings are routinely performed 24-hours per day and 7 days per week until sufficient seizures are recorded. This usually necessitates a mean recording duration of 5 to 14 days. The presence of EEG technologists throughout the course of intracranial EEG study is necessary to insure the technical integrity and high quality of the EEG recording. Patient care is provided by a well-trained medical staff, who can provide a neurological evaluation to test awareness, language, memory, and sensory-motor functions during and after a seizure. It is also important to have continuous supervision to prevent the patient from falling or pulling out intracranial electrodes during or after a seizure.
EEG Acquisition
Methods for intracranial EEG recording are essentially identical to those used for scalp EEG. Data are routinely digitized at 200 to 250 Hz, which provides an accurate representation of common clinical EEG frequencies, such as delta, theta, alpha, beta, and gamma. For these routine studies, band-pass filters are typically set at 0.3-Hz low-frequency filter and at 70-Hz high-frequency filter. However, modern commercial amplifiers have the ability to sample at much higher rates, ranging from 1,000 to 2,000 Hz. This allows the faithful recording of high-frequency EEG patterns, such as high-frequency oscillations (HFOs) (ripples and fast ripples) from 80 to 500 Hz. Obviously, to record these patterns, the high-frequency filter needs to set much higher, such as at 500 Hz.
The number of recording channels required is dependent on the number of implanted subdural electrodes. Although contemporary commercial EEG equipment can simultaneously record 128 to 256 channels, many routine clinical intracranial EEG studies may only require 50 to 100 channels. EEG channels are generally displayed in a logical montage according to anatomic distribution (e.g., anterior to posterior, superior to inferior). EEG data can be reviewed in either referential or bipolar montages. Each has advantages and disadvantages.
Referential montages display the absolute voltage perceived by an electrode contact, either from a near-field or far-field generator, relative to that of the reference. Because subdural electrodes are placed on the cortex, they record exclusively from gray matter, and near-field signals from underlying cortex predominate. However, in referential recordings from depth electrodes in white matter, there is no local EEG generator, so far-field signals predominate. Referential recordings can be subject to a variety of external artifacts. Therefore, it is important to choose a reference electrode that has minimal signal, less likelihood of artifacts, and is less likely to be involved in seizure activity. Either intracranial or extracranial electrodes can be used as the reference. Intracranial references are less influenced by external movement and muscle artifacts, but unless properly chosen, they may record a significant amount of epileptiform activity, thus potentially confusing the EEG interpretation. Accordingly, intracranial contacts not touching the cortex and distant from likely epileptic sources are typically used for reference. The amplitude of EEG signals recorded at the scalp is attenuated compared to that from the cortex, so a scalp electrode may be a good reference for intracranial EEG. For example, vertex scalp electrodes (such as CPz) are a good choice for reference when evaluating TLE because they record little temporalis muscle artifact and are distant from temporal lobes. For opposite reasons, earlobe electrodes A1 or A2 would not be recommended.
Bipolar montages with subdural electrodes display the difference in voltage typically between adjacent electrodes on a strip or grid. Very focal, near-field activity is accentuated, whereas broad near-field and far-field activity is suppressed. Thus, little or no interictal or ictal potentials may be evident in a channel composed of recordings from two highly, but similarly, active electrodes. Bipolar montages may be more useful in depth electrode recordings where there are few contacts in any given area of cortex, so in-phase cancellation is minimized. This lack of signal on a bipolar depth electrode channel may however be useful in identifying white matter because the far-field signals from the distant cortex will be suppressed. Accordingly, it is important to review intracranial EEG data with both referential and bipolar montages.
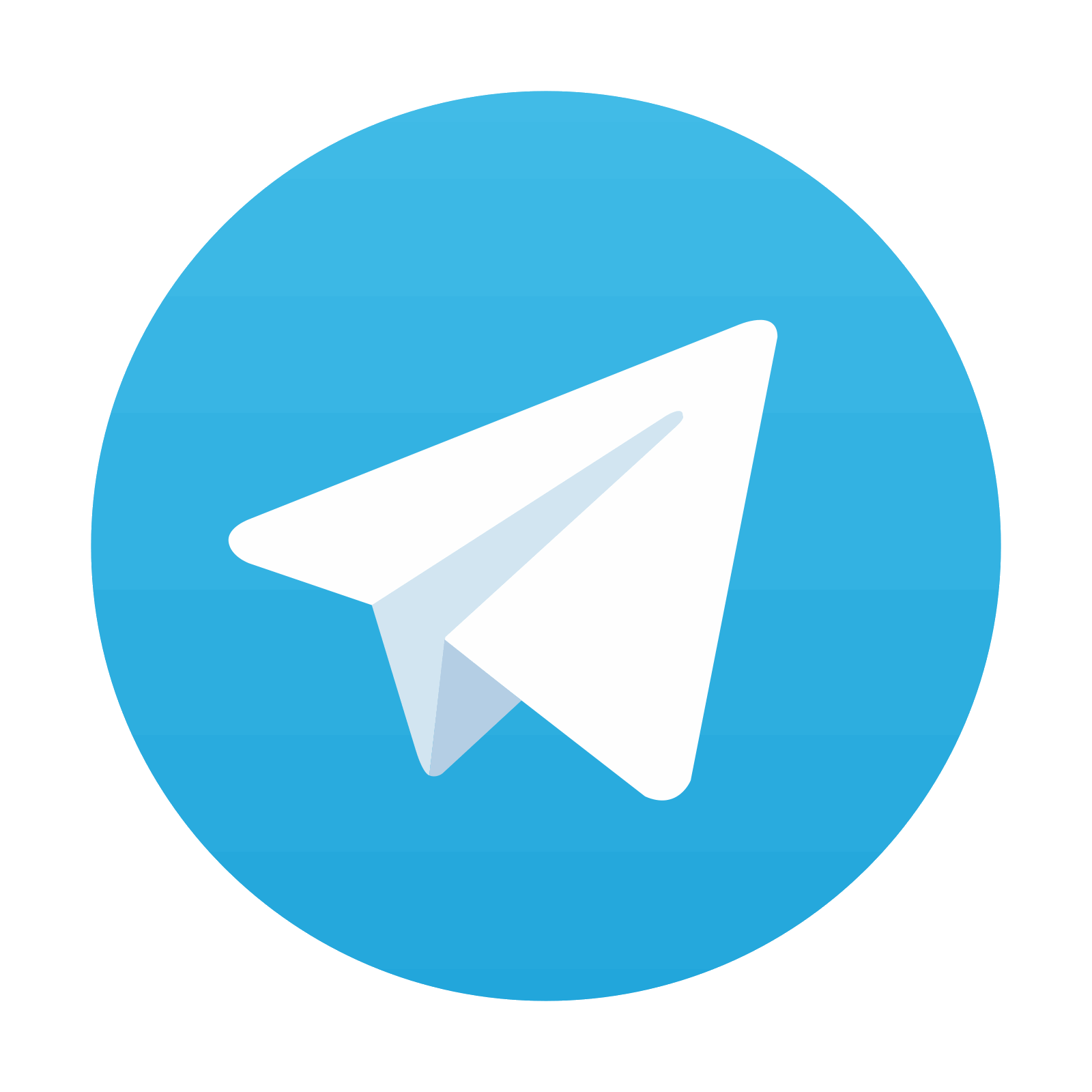
Stay updated, free articles. Join our Telegram channel
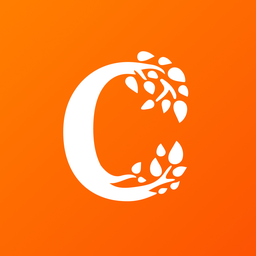
Full access? Get Clinical Tree
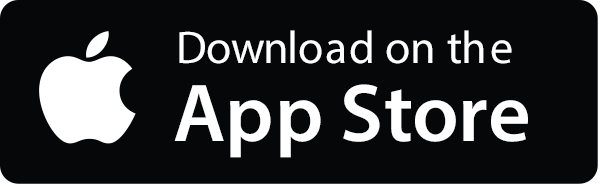
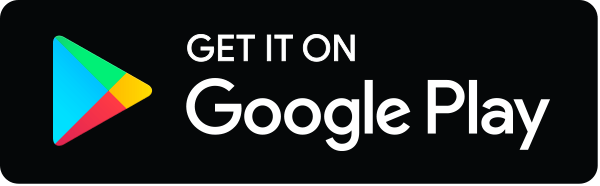