The prominent roles of the lungs and the kidneys in acid-base homeostasis were described in Chapter 8. In this chapter, we take a more in-depth look at precisely how the lungs and the kidneys perform these functions. Regarding the regulation of volatile acid, some of the major factors that control and regulate ventilation in health and disease are reviewed. The control of ventilation is a complex physiologic process. The major factors that play a role in the regulation of ventilation are shown in Figure 12-1. The primary respiratory center (generator) is located in the medulla of the brain (medullary center). Output of the medullary center is influenced by several other centers in the brain that affect respiration. The apneustic and pneumotaxic centers in the pons tend to modify the ventilatory pattern, and the cerebral cortex may participate in voluntary input into the system. The CSF is separated from the blood by the blood-brain barrier, which is readily permeable to gases but relatively impermeable to ions. Gases equilibrate quickly across the blood-brain barrier. Some ions, such as bicarbonate, may tend to equilibrate across the barrier, but the exchange process is active transport rather than simple diffusion. The active transport of ions across the blood-brain barrier may take a considerable time (i.e., hours to days)81 compared with the immediate diffusion of gases. Cheyne-Stokes breathing is a recurrent pattern of ventilation characterized by a progressive rise and fall of tidal volume (Fig. 12-2). A period of apnea may sometimes occur between cycles. The related alveolar and central chemoreceptor PCO2 levels at different points in the breathing cycle are also shown in Figure 12-2. Unlike the central chemoreceptors, the peripheral chemoreceptors respond to several different blood gas stimuli: the PaO2, arterial pH, and PaCO2. In addition, when blood flow past the peripheral chemoreceptors is diminished (e.g., in shock), an increase in ventilation is also stimulated. The peripheral chemoreceptors generally do not respond to anemia (e.g., methemoglobinemia, HbCO poisoning), although there is some response when anemia is severe. Interestingly, the peripheral chemoreceptors stimulate severe hyperpnea (increased tidal volume) in cyanide poisoning.81 Although both the peripheral and central chemoreceptors respond to increased PaCO2 and decreased pH, they are not equally sensitive to these stimuli. Specifically, a relatively large increase in PaCO2 or a decrease in pH (e.g., PaCO2 increase = 10 mm Hg; pH decrease = 0.1)81 is necessary before a notable increase in ventilation will be triggered via the peripheral chemoreceptors. Conversely, the central chemoreceptors respond to very slight changes in PaCO2. In a normal young man, minute ventilation increases approximately 2.5 L with only a 1-mm Hg increase in PaCO2.81 In chronic hypoxemia, PaCO2 also remains low. After a few hours, however, the pH of the CSF, made alkalotic by the hypocarbia, begins to return to normal as bicarbonate ions are actively transported out of the blood-brain barrier. When the pH of the CSF returns to normal, it no longer depresses ventilation via the central chemoreceptors. At this point, the individual actually begins to hyperventilate to a greater degree. It is, in fact, well known that the ventilatory response to chronic hypoxemia is greater than the ventilatory response to acute hypoxemia because of this mechanism.81 Again, the ventilatory pattern at any point in time depends on the interaction of the chemoreceptors. The effects of progressive pulmonary deterioration on the chemoreceptors and blood PaCO2 and PaO2 levels are shown in Table 12-1. Normal lung function is associated with normal blood gases and ventilation is primarily under the control of the central chemoreceptors. Table 12-1 Regulation of Ventilation and Blood Gases in Progressive Pulmonary Disease The initial blood gas abnormality associated with mild pulmonary disease is mild hypoxemia with a normal PaCO2 (see Table 12-1). In this early stage, the increase in peripheral chemoreceptor stimulation is so minute that it is not clinically detectable. The central chemoreceptors maintain primary control over ventilation. As deterioration in external respiration continues, PaO2 continues to decline. At a PaO2 level of approximately 60 mm Hg (although there may be considerable individual variation with regard to the specific PaO2 when this occurs), a dramatic increase in peripheral chemoreceptor stimulation is seen, and the peripheral chemoreceptors assume primary control of ventilation. The strong peripheral chemoreceptor drive usually results in an increase in alveolar ventilation and a fall in the PaCO2 (see Table 12-1). If external respiration continues to deteriorate, CO2 excretion is ultimately impaired and PaCO2 levels begin to increase. Furthermore, PaO2 levels continue to fall (see Table 12-1). Indeed, the classic definition of acute respiratory failure is a PaCO2 greater than 50 mm Hg and/or a PaO2 less than 50 mm Hg. The same pattern of progressive pulmonary deterioration can also occur over a short time (days or hours) in acute pulmonary disease. This pattern may be observed in pneumonia, postoperative respiratory failure, or acute asthma. It is always important to identify patients with moderate impairment (i.e., moderate disease as described in Table 12-1), because further deterioration leads to hypercarbia. The classic example of this is the patient in status asthmaticus (sustained unresponsive asthma) whose condition deteriorates progressively over a period of days, leading ultimately to exhaustion and to the abrupt onset of respiratory acidemia. Other studies have shown that the worsening hypercarbia associated with oxygen therapy in these patients is more likely a result of ventilation-perfusion alterations than a result of a decrease in ventilatory drive.463 Furthermore, the Haldane effect (release of CO2 from Hb into the blood in the presence of increased oxygen) may be responsible for some of the ensuing hypercarbia.464 The precise mechanism responsible for this hypoventilation remains a controversial issue and multiple factors may be influencing ventilation simultaneously. Regardless of the exact mechanism, worsening hypercarbia must be recognized as a possible consequence of oxygen therapy in chronic lung disease. At least six different reflexes have been described in relation to the regulation of ventilation.81 The precise role of many of these reflexes must still be defined. Nevertheless, two reflexes may be useful in helping the clinician to understand the origin of respiratory alkalosis in certain pulmonary conditions. The gross anatomy of the kidney is shown in Figure 12-3. Each of the two kidneys consists of an outer cortex and an inner medulla. Urine formed in the functional units of the kidney gathers in the renal pelvis and then flows through the ureters down to the urinary bladder, where it is stored. Ultimately, urine is excreted through the urethra. The functional unit of the kidney is the nephron. Each kidney contains approximately 1 million nephrons. A schematic drawing of the functional nephron is shown in Figure 12-4. Blood enters the nephron through the afferent arteriole, which in turn enters an enclosed capsule. This capsule, called Bowman’s capsule, is actually the first portion of the renal tubular system. The volume of glomerular filtrate formed depends on the volume of renal perfusion. Normally, the kidneys receive approximately 20% of the cardiac output. The amount of this volume that is filtered out into the glomerular filtrate is also large. A volume roughly equivalent to the entire extracellular fluid volume (i.e., 15 L) passes through the glomeruli every 2 hours.465 In the patient with reduced volume and metabolic alkalosis, glomerular filtration is likewise reduced. This perpetuates the syndrome of metabolic alkalosis as excess [HCO3] cannot be excreted.480 Thus, correction of metabolic alkalosis is dependent on adequate renal perfusion and glomerular filtration. Approximately 99% of all the fluid that passes into the glomerular filtrate is reabsorbed. As the filtrate passes through the nephron, various electrolytes and substances are reabsorbed in proportion to the body’s needs. As shown in Figure 12-4, a rich supply of capillaries (i.e., peritubular capillaries and vasa recta) is immediately adjacent to the renal tubules that facilitate reabsorption of many of these electrolytes back into the bloodstream. Approximately 60% of the body’s weight is made up of water. This water is separated by membranes into various body fluid compartments (Fig. 12-5). As further shown in Figure 12-6, approximately two-thirds of the water (approximately 65%) is located in the intracellular fluid space, or within cells. The remaining 35% exists in the extracellular fluid compartment or outside the cells.
Regulation of Acids, Bases, and Electrolytes
OVERVIEW
REGULATION OF VENTILATION
Chemoreceptors
Central Chemoreceptors
Blood-Brain Barrier
Cheyne-Stokes Ventilation
Peripheral Chemoreceptors
Responsiveness
PaCO2/pH
Chemoreceptor Interactions
Chronic Hypoxemia
Normal Lung Function
Progressive Disease
Normal
Mild
Moderate
Severe
Blood gases
PaO2
100 mm Hg
65 mm Hg
55 mm Hg
50 mm Hg
PaCO2
40 mm Hg
40 mm Hg
34 mm Hg
50 mm Hg
Central chemoreceptors
+ + + +
+ + + +
+ +
+
Peripheral chemoreceptors
+
+ +
+ + +
+ + + +
Control
C
C
P
P
Mild Disease
Moderate Disease
Severe Disease
Reflexes
RENAL FUNCTION
Macroscopic Anatomy and Physiology
Microscopic Anatomy and Physiology
Urine Formation
Glomerular Filtration
Tubular Reabsorption
BODY FLUIDS AND ELECTROLYTES
Fluid Compartments
< div class='tao-gold-member'>
Stay updated, free articles. Join our Telegram channel
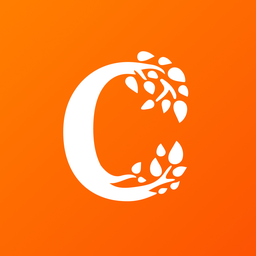
Full access? Get Clinical Tree
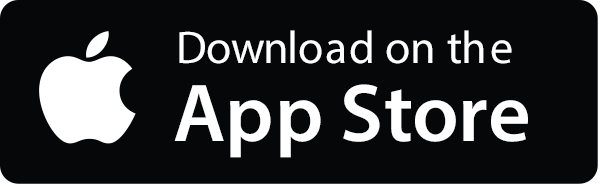
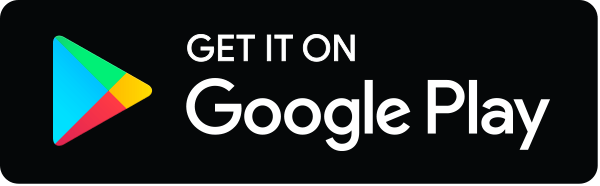