Fig. 17.1
The stages of tracheal and lung development in human and mouse. Genetic regulation of mouse tracheal specification from foregut endoderm (Bmp4) and patterning (Shh) is indicated in bottom left panel. Lung branching regulation by Fgf10 from mesenchymal side and Shh, Spry2, Tgfb, and Dicer from epithelial side is specified in bottom middle panel. Lung vascularization by Vegf-a from epithelial cells and lung septation regulation by Hgf, Fgf1,7 from mesenchymal and endothelial cells is indicated in bottom right panel
At different stages, respiratory tissue specification is predominantly regulated by the cellular compartment, relying on epithelial–mesenchymal crosstalk [7]. It involves interactions between different cell representatives of lung epithelium and mesenchyme by gene expression and paracrine effects, extensively studied in mouse models. The dorsal-ventral endodermal patterning during primordial bud separation is controlled by bone morphogenetic protein 4 (Bmp4) signaling in mouse [8] (Fig. 17.1). Differentiation of tracheal epithelial cells (ciliated, non-ciliated secretory, basal, and small mucus granule cells) occurs in a polarity-based cartilage-oriented manner [9]. The development and patterning of C-shaped hyaline cartilage rings in the trachea is regulated by sonic hedgehog (Shh) expression [10] (Fig. 17.1). The rings are connected by smooth muscle on the posterior side. The ECM defines the unique mechanistic properties of the trachea which are critical for bioengineering the organ.
In the lung , the branching of the bronchial tree was demonstrated to be controlled by fibroblast growth factor 10 (Fgf10) expressed in mesenchymal cells [11, 12], and Sonic hedgehog (Shh), Sprouty2 (Spry2) and Dicer1 expressed in epithelial cells during pseudoglandular stage [13–16] (Fig. 17.1). At later stages, alveolar wall remodeling involves redistribution of tissue mass and stretching of septa initiated by the proliferation of interstitial fibroblasts regulated by PDGF-A [17]. Further proliferation of AEC-II is known to be triggered by the factors released by endothelial and mesenchymal cells: fibroblast growth factors 1 and 7 (FGF1, FGF7), hepatocyte growth factor (HGF), and retinoic acid (RA, the active metabolite of vitamin A) [18–20] (Fig. 17.1). Transforming growth factor beta (TGF-β) is also hypothesized to be involved in AEC differentiation [21]. Respiratory blood vessel formation is highly dependent on vascular endothelial growth factor A (VEGF-A), produced by AECII [20, 22] (Fig. 17.1). Inhibition of VEGF receptors causes both alveolar and endothelial cell apoptosis and enlargement of lung airspaces [23], which suggests that lung alveolar maintenance is highly dependent on the VEGF pathway. The knowledge gained from understanding the roles of different regulatory pathways and paracrine factors or chemical agents (e.g., dexamethasone, RA [24, 25]) that could affect lung development and structure formation, are valuable for the field of regenerative medicine studies.
Endogenous Progenitor Stem Cells
Progenitor and stem cells are involved in respiratory tissue regeneration and homeostasis in the respiratory system in cases of injury or disease. Various cell types can be affected by majority of lung diseases, and therefore different progenitor cell types would be involved in restoration and repopulation of the trachea and lung. Apart from epithelial cells, there are also interstitial fibroblasts, vascular, lymphatic and neuronal cells that might be damaged by respiratory disease s, with ECM also being affected. There are endogenous and exogenous sources of stem cells for the lung [26]. Among endogenous epithelial stem cells there are basal epithelial cells, variant club cells, bronchoalveolar stem cells, and AEC-II, that are hypothesized to be precursors of most lung epithelial cells [27]. Recent studies indicate that endogenous progenitors also include side population (SP) cells with mesenchymal (chondrocyte, osteocyte, and adipocyte), endothelial, and potentially epithelial differentiation capacity [28–30]. Isolation of endogenous cells from donors for therapeutic and bioengineering purposes is rather complicated since they are not easily accessible; therefore leading to the consideration of exogenous stem-cell sources as an alternative.
Exogenous Progenitor and Stem Cells
Prospective exogenous stem-cell treatments have to target regeneration of ECM and the multiple lung cell types (including epithelial, mesenchymal, and endothelial) that can be affected in respiratory disease s. There are several donor tissues e.g., bone marrow (BM), peripheral blood (PB), amniotic fluid (AF), or umbilical cord blood (UCB) that could serve as sources of exogenous stem and progenitor cells , including endothelial and mesenchymal progenitor cells [26]. Bone marrow, PB, AF, and UCB were reported to contain progenitor cells for blood, endothelium, and mesenchyme, which can contribute differently to the respiratory tract regeneration (Table 17.1) [31–36]. AF-derived stem cells were demonstrated to possess epithelial differentiation potential [37–39]. Cells from all these sources exhibit reparative properties in various lung disease models [37, 38, 40–44]. They can be involved in the regeneration process directly (proliferating in vivo, differentiating towards the targeted cell type at the injury site, or modulating ECM, see Table 17.1) or indirectly (contributing to lung homeostasis via epithelial–mesenchymal crosstalk) [45]. This involvement suggests that donor-derived stem cells can be a suitable tool for understanding the mechanism of injury repair [46–48], and offer possibilities in therapeutic application.
Table 17.1
Examples of exogenous stem cells that can contribute to lung regeneration
Stem cells | Source | Cell fate | Isolation | Effects in lung | Reference |
---|---|---|---|---|---|
MSC | BM, PB, UCB, placenta, adipose tissue | Bone, cartilage, fat, fibroblasts | Biopsy or blood donation, high numbers in donated tissues, distinguished cell population | Contribute to fibrocytes, support ECM, involved in immunomodulation | |
EPC | BM, PB, UCB | Endothelium | Biopsy or blood donation, low numbers in donated tissues, no uniform markers for isolation | Contribute to vascularization | |
HSC | T cells and B cells, monocytes, macrophages, neutrophils, eosinophils, mast cells, erythrocytes, megakaryocytes | Biopsy or blood donation, high numbers in donated tissues, distinguished cell population | Contribute to blood pool | ||
AFSC | Amniotic fluid | Multipotent (including MSC and EPC), epithelial | Amniocentesis (potential side effects to donor), multiple cell types | Contribute to bronchial and alveolar epithelial cells |
Tissue Engineering
The key elements to consider in TE are the production of a suitable biocompatible matrix that preserves spatial geometric architecture, recapitulates the biomechanical properties of the native tissue, and also retains bioactive cues. The matrix can be based on biological tissue or synthetic materials. In the case of biological matrices, donor tissue is treated and/or manipulated via various methodologies aiming for the removal of antigenic components. This so-called decellularization process is highly critical since it determines the characteristics of the matrix and the fate of the seeded cells.
Various physical methods (perfusion, agitation, static, sonication), different flow rates, application temperature, different reagents (DNase, sodium deoxycholate, Triton-X, sodium dodecyl sulfate (SDS), (3)3-[(3-cholamidopropyl) dimethylammonio]-1-propanesulfonate (CHAPS)) and processing period are used to rebuild de novo tissues and organs that are non-immunogenic. This is clinically important for use in transplantation medicine. Although unlike biological matrices that retain various bioactive characteristics due to preservation of ECM, synthetic materials may also serve as basic scaffolds in TE.
Synthetic scaffolds can be made from biodegradable or non-degradable materials, and engineering procedures range from moulding to electrospinning, 3-D printing, and others. Because synthetic scaffolds are not derived from donor tissue, they do not pose a contamination risk during development , and can be rapidly produced and reproduced using relatively economical engineering processes. On the other hand, biological scaffolds hold significant bioactive information and cues that are important for the correct engraftment, proliferation, and differentiation of seeded cells. Because these cues are entirely lacking in synthetic scaffolds, this complicates their use in vivo or in clinical translation. New strategies and concepts are required to overcome these hurdles. The design of synthetic matrices using nanotechnology approaches may open a new direction in this so-far dead-end street of synthetic scaffolds.
Tracheal Bioengineering
Disorders affecting more than 50 % of the entire length of the adult trachea (or 1/3 in children) are inoperable due to the lack of reconstructive tissue. During the last decade, various surgical techniques and experimental methods have been investigated [49]. All of them have eventually failed due to time consuming protocols, heavy immunosuppression, or extremely difficult surgical procedures. Tissue engineering may provide a potential solution to this challenging situation. Based on experimental in vitro and in vivo animal data, the first-in-man transplantations of tissue-engineered tracheae have recently been performed, using both decellularized and synthetic scaffolds [50, 51]. The early and intermediate success, recently published in our 5-year-follow-up demonstrates the feasibility of this methodology [52]. Long-term data and clinical trials will provide significant data that may prove the real clinical meaning of this novel, currently rather conceptual strategy for tracheal replacement and reconstruction. Currently, a number of clinical as well as experimental research groups are investigating the use of both biological decellularized and synthetic tracheal scaffolds [50, 51, 53, 54]. A report from the recent expert group meeting [55] came to the conclusion that at present there is no consensus with regard to treatment methods for patients that suffer from complex tracheal disorders (Table 17.2). Even though the trachea appears to be a simple tube, its reconstruction or replacement remains challenging. Thus, research regarding novel methods of tracheal tissue regeneration , cell–surface interactions, and in vivo transfers are necessary to advance clinical frontiers in this challenging field.
Table 17.2
The clinical experience of transplanting biological and synthetic tracheae
Method | Method | Pros & Cons | Application field |
---|---|---|---|
Allotransplantation | Donor organ | ||
All donor and the majority stent dependent | Fresh organ | Pros: processing time | Some few reports in human |
Cons: immunosuppression needed, surgically challenging | |||
Cryopreserved, irradiated | Pros: no need for immunosuppression | Some few reports in human | |
Cons: initial stenting | |||
Chemical fixation | Pros | – 100 Adults | |
– No need for immunosuppression | – 31 Children | ||
Cons | – Only one cancer patient | ||
– Not applicable for circumferential replacement | |||
– Relies on local reconstructive tissue | |||
– Long processing | |||
– Permanent stenting | |||
Fresh aortic allograft | Pros: processing time, no need for immunosuppression | Initial clinical application in six human cases | |
Cons: requires permanent stenting | |||
Composites | In vivo allograft epithelialization | Pros | One clinical case |
– No ex vivo culture | |||
Cons | |||
– Relies on a donor trachea | |||
– Processing time | |||
– Initial immunosuppression | |||
Polypropylene mesh covered with collagen sponge | Pros | Initial clinical application (patch) | |
– No need for immunosuppression | |||
Cons | |||
– No long segments | |||
Tissue-engineered transplantation | Biological scaffold | ||
Decellularized human trachea | Pros | Various patients with circumferential and partial replacement in both benign diseases and malignancies | |
– No need for immunosuppression | |||
– Biodegradable | |||
– Maintained ECM | |||
– Cell homing | |||
– Biocompatibility | |||
– Proangiogenicity | |||
Cons | |||
– Donor dependent | |||
– Ex vivo processing | |||
– Stenting in the intermediate and long-term follow up | |||
Decellularized porcine Jejunum | Pros | Three clinical cases (Patch), treating malignancies | |
– Biocompatibility | |||
Cons | |||
– Mechanical strength | |||
– Shortage of donor | |||
– Processing time | |||
– Immunogenicity? | |||
Synthetic scaffold | |||
POSS covalently bonded to PCU | Pros | One clinical case (circumferential entire trachea + bifurcation) | |
– Customized | |||
– Rapid and cheap production | |||
Cons | |||
– Lack of proangiogenic factors | |||
– Mechanical properties | |||
– Tissue integration | |||
PET/PU (nanofiber) | Pros | Various clinical cases with different outcomes | |
– Customized | |||
– Mimicking ECM structure | |||
– Allows for cell adhesion and migration | |||
– Rapid and cheap production | |||
Cons | |||
– Lack of pro-angiogenic factors | |||
– Mechanical properties |
Lung Bioengineering
Bioengineering of the lungs is complex, since this organ contains an intricate structure and a plethora of functions. There are over 60 different pulmonary cell types with multifaceted functions, an intricate alveolus-capillary interface to maintain gas exchange, and a fine network of microvasculature that must resist thrombosis and preserve elasticity for lung compliance to be adaptable for pressure–volume changes [56, 57]. One of the major challenges for bioengineering a three-dimensional lung structure is preserving or re-creating a delicate ECM with elasticity required for alveolar expansion, and thinness for efficient gas exchange.
The ECM microenvironment has a prominent role in identifying putative lung progenitor cells and driving cellular fate to maintain tissue integrity [58–60]. In turn, the composition of ECM is also highly dependent on, and can be manipulated by, the surrounding cell types. The non-alveolar lung tissue consists of approximately 50 % ECM proteins [58]. The predominant ECM proteins, collagens, and elastin fibers provide structural and mechanical strength while laminin, fibronectin, and glycosaminoglycans (GAGs) are essential for cell attachment, proliferation, and differentiation [61–63]. However, the composition of ECM is highly dependent on the applied decellularization method [64–66]. Aside from preserving ECM composition, an ideal decellularization protocol should also preserve the gross native architecture of the lung (Fig. 17.2) that allows the scaffold to be physiologically ventilated and perfused [65]. Early experimental in vivo data do suggest that even complex structures such as the lung can be engineered. However, these experiments were performed in immunodeficient rodent models that cannot be immediately translated into a clinical setting because they do not address the most relevant clinical questions and concerns about graft rejection and the need for immunosuppression. Nonetheless, this type of experiment using decellularized lung tissues can be used to investigate and further understand various pulmonary cell–surface interactions, physiological changes, cell fate, and organ development . Besides, TE technologies can be applied to investigate lung disease models, such as elastase or bleomycin-induced lung injuries and the underlying pathohistological changes in the ECM [59, 67].
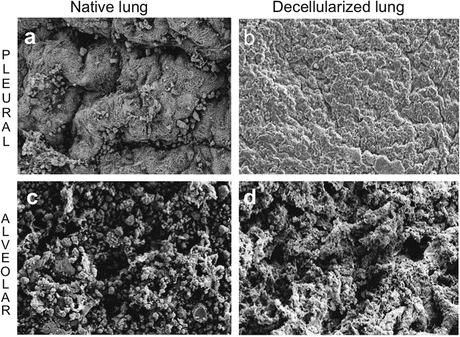
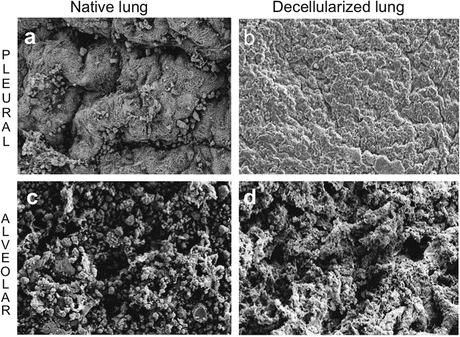
Fig. 17.2
An ideal decellularization protocol maintains gross native architecture. Scanning electron microscopy evaluation of pleural (a, b) and alveolar (c, d) lung surfaces before and after decellularization with 1 % deoxycholate and 0.1 % Triton-X-100. Scale bar 20 μm
In Vitro Bioassay Systems
In vitro assays using decellularized lung scaffolds [68, 69], 2-D monolayer culture systems [70], or 3-D matrices such as matrigel [71], or porcine skin gelatin (Gelfoam) [72] have been used to assess the reparative and regenerative capacity of candidate endogenous lung stem cells and exogenous stem cells. Huh and colleagues [73] have developed a lung-on-a-chip, to create a combination of different cell types and a mechanical force interface to simulate living organs. Biomimetic microsystems of this type are useful to determine if implanted cells can release therapeutic and immunomodulatory substances at an adaptive dynamic rate, as judged by the cellular feedback mechanism. This can also be an accurate alternative to “replace, reduce, and refine” animal studies for predicting lung responses [73].
Ex Vivo Bioreactor System
Numerous research groups have successfully utilized three-dimensional models (trachea and en bloc lung ) to characterize biocompatibility and biomechanics after tissue decellularization and recellularization. These models can potentially be used as tools for researching disease models, cell therapy , cell differentiation and fate, and drug application. The choice of stem cells for recellularizing tissues or organs has been a major focus of investigation in TE [74]. Cell types such as embryonic [75, 76] and induced pluripotent stem cells [68], amniotic fluid stem cells, mesenchymal stromal cells [77] or peripheral blood and bone-marrow-derived mononuclear cells are currently under investigation for their potential uses in different models using mouse, rat, porcine, non-human primate and human cadaveric donors. Furthermore, these ex vivo systems enable one to create simple tools to examine microenvironmental variables such as scaffold stiffness, mechanical ventilation, growth factors , pH, etc. and influence cell adhesion, proliferation, differentiation, or transdifferentiation [60, 78–80] (Fig. 17.3). These systems will also help to identify those factors that are important for providing the complex structural and biophysical cues required for tissue remodeling and regeneration .
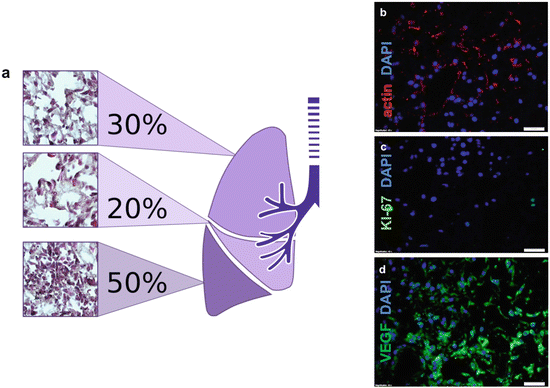
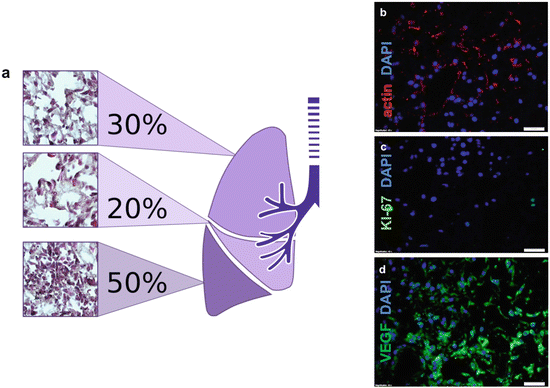
Fig. 17.3
Recellularizing ex vivo lung scaffold with plastic adherent bone-marrow-derived MSCs (BM-MSCs). Cells at passage 5 were delivered via pulmonary artery perfusion at 1 mL/min in a bioreactor for 7 days and were evaluated. (a) Cellular biodistribution on whole organ scaffold with bone-marrow-derived plate-adherent stem cells . Haematoxylin and eosin staining indicates the presence of reseeded cells on the scaffold. The lower lobe has the highest (50 %) number of detected cells. (b–c) Cell-to-matrix characterization of recellularized lung; (b) cytoskeleton is stained using actin antibody in red, (c) cell proliferation is indicated using Ki-67 antibody in green and (d) vasculogenesis marker VEGF presence is indicated in green. Scale bar 20 μm
Tumour Initiating Cells in the Respiratory Tract
In some circumstances, patients who opt for potential therapeutic options utilising cell therapies or tissue-engineered scaffolds (e.g., tissue-engineered tracheal replacements) could suffer from recurring tumours. According to the cancer stem-cell paradigm it is believed that dysfunctional resident stem-like cells undergo oncogenic transformation giving rise to tumor-initiating cells which generate benign or malignant tumors [81]. Activated cancer-associated fibroblasts are also an integral component of the tumor microenvironment , influencing tumor growth and progression (e.g., by secreting growth factors , altering tissue metabolism and remodeling ECM) [82, 83]. Experimental models for the analysis of the interaction of airway tumor initiating cells with biomatrix scaffolds are essential in order to devise optimal therapeutic protocols employing tissue engineering technologies in lung cancer patients. Although various mouse models and assays have been used to identify adult lung stem and progenitor cells , and analyze their behavior in the normal and diseased lung [45, 84–86], the identity and properties of human airway stem/progenitor cells and tumor initiating cells are less clear.
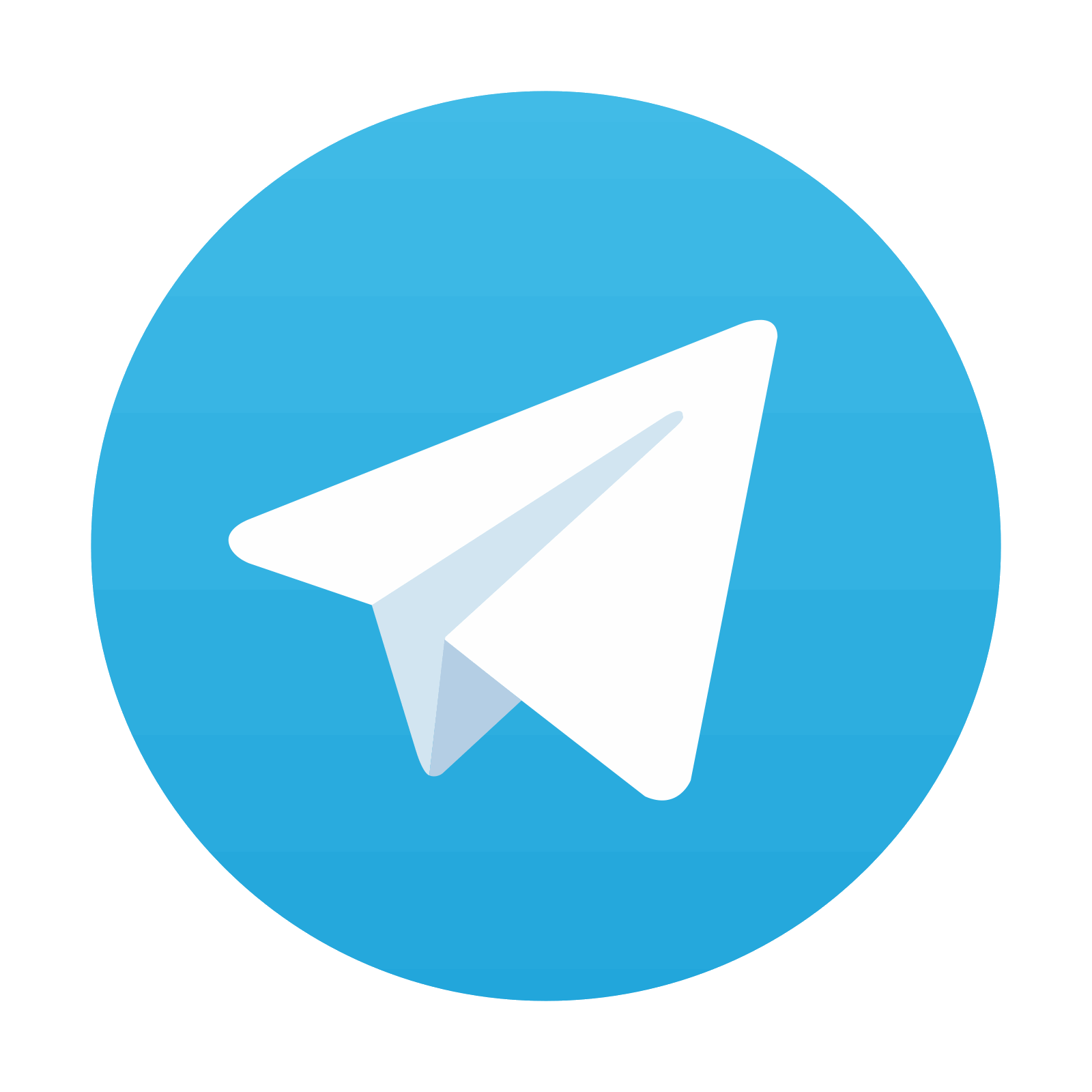
Stay updated, free articles. Join our Telegram channel
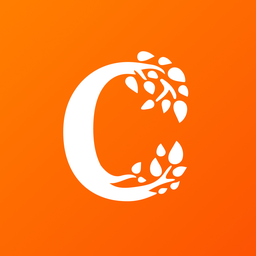
Full access? Get Clinical Tree
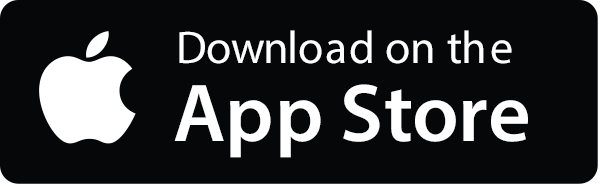
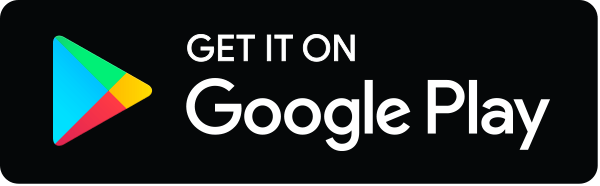