Fig. 18.1
Schematic of ex vivo organ engineering. Autologous cells are obtained by a biopsy from the eventual transplant recipient and expanded in ex vivo culture. A scaffold, either synthetic or an acellular lung, is manufactured and repopulated ex vivo by the usage of a bioreactor to create a functional tissue suitable for re-implantation
An exciting new and active area of research involves the use of acellular lung scaffolds derived from cadaveric or failed transplant lungs. Acellular tissue is generated by removing cells from the native organ while preserving the 3D macroarchitecture and the majority of the extracellular matrix (ECM) proteins [5–17]. Whole organ decellularization as a platform for organ regeneration was first described in the heart in 2008 [15] and beginning in 2010, several groups described similar techniques in lung [18–23]. The use of acellular lungs has since expanded beyond their usage in regenerative medicine and has become an incredibly powerful in vitro tool for studying cell–ECM interactions or the impact of diseased matrix on cellular behavior [24–29].
This chapter discusses the status of current areas of research investigating ex vivo regeneration of lung tissue, and includes a discussion of concepts learned from the literature on ex vivo tissue culture and organ preservation.
Engineering a Scaffold
Designing and Manufacturing a Bioartificial Scaffold
Proposed bioartificial scaffolds for lung engineering have been manufactured by various techniques and from a variety of different materials. An overview of the current approaches is given in Table 18.1. In general two different methods of scaffold generation can be distinguished: additive (layer-by-layer or unit-by-unit generation) and subtractive methods (generation by removing material to form the final scaffold). Additive techniques benefit from the possibility to generate scaffolds with interconnecting pores. Depending on the resolution needed, however, these techniques may lead to long fabrication times. Examples for additive techniques are rapid prototyping and 3D bioprinting [30]. Subtractive methods such as porogen forming techniques and sphere-templating have also shown promising initial results [31, 32] but are more limited regarding scaffold design. There are various other methods to produce scaffolds for tissue engineering purposes like solvent casting, particulate leaching, melt molding, or freeze drying. Synthetic materials used thus far are polymers like polyglycolic acid (PGA), poly-lactic-co-glycolic acid (PLGA), poly-l-lactic-acid (PLLA), polyurethane (PU), and polyvinyl (PV) in order to match the mechanical properties of lung tissue. Hydrogels made of collagen I, gelatin, Matrigel, alginate, fibrinogen–fibronectin–vitronectin combinations or PGA combined with Pluronic F-127 have been used as scaffolds as well [33, 34]. Further, synthetic scaffolds can be loaded with growth factors, ECM components (e.g., collagen or whole lung extracts) or peptide sequences known to facilitate cell attachment (e.g., RGD) [35].
Table 18.1
Artificial scaffolds used for Tissue Engineering of the lung
Ref. | Scaffold | Study objective | In vitro/in vivo | Cell source seeding | Benefits/limitations |
---|---|---|---|---|---|
[83] | Polyglycolic acid and Pluronic F-127 hydrogel | Isolation and characterization of somatic lung progenitor cells and the promotion of alveolar tissue growth | In vitro, in vivo | Somatic lung progenitor cells | De novo generation of lung tissue, limited amount of cell source |
[118] | PDLLA scaffolds (3D foam) | Suitability of scaffold for distal lung tissue engineering | In vitro | MLE-12 | No toxic effects, supports cell growth |
[119] | Matrigel, synthetic poly-lactic-co-glycolic acid (PLGA) and poly-l-lactic-acid (PLLA) porous foams and nanofibrous matrices | Engineering of 3D pulmonary tissue constructs | In vitro | Murine embryonic day 18 fetal pulmonary cells | Limited amount of cell source; use of mixed populations |
[120] | Collagen I gel | Effects of FGF10, FGF7, and FGF2 on murine fetal pulmonary cells to control epithelial cell behavior | In vitro | Fetal murine pulmonary cells | Addition of growth factors to influence cell proliferation and differentiation possible |
[84] | Gelfoam sponge (collagen I) | Application for cell-based lung regeneration | In vivo | Fetal lung cells (d19) | No severe local inflammatory response, sponge degraded after several months |
[31] | Patterned porous hydrogel from poly(2-hydroxyethyl methacrylate) (poly(HEMA)), Collagen I treated | Suitability of scaffold fabrication method to design scaffolds with different macro- and microstructure, cell attachment | In vitro | Mouse skeletal myoblast cells | Tailoring of scaffold macro- and microstructure possible |
[85] | Matrigel plug combined with FGF2-loaded polyvinyl sponges | Generation of vascularized pulmonary tissue constructs | In vivo, subcutaneous | Fetal pulmonary cells (d17.5) | limited amount of cell source, induction of a host inflammatory response |
[35] | Fibrinogen–fibronectin–vitronectin hydrogel | Ability of hydrogel to promote cell engraftment | In vivo | Primary ovine lung mesenchymal cells | Mechanical properties similar to lung, safe cell delivery, no atelectasis and scarring |
[121] | Cylindrical-shaped bronchioles, fibroblasts embedded into collagen I with ASM cells on the outer surface, epithelial cells on the inner surface (lumen) | Development of a tissue-engineered bronchiole | In vitro | Human lung primary cells (fibroblasts, airway smooth muscle and epithelial cells) | Cell–cell interactions and airway remodeling events can be studied |
[74] | Cellularized collagen matrix (Gelfoam) | Treatment of postpneumonectomy space | In vivo | BMSC | Cells only survive in early time points inside the scaffold (until d7) |
[34] | Nanofibers coated with lung extracts | Effect of lung extract and modulus on cell differentiation | In vitro | Bone marrow cells | High throughput possible |
[33] | Collagen-elastin hydrogels | Effect of elastin and cell seeding on hydrogel stiffness, Suitability as building blocks for lung engineering | In vitro | Lung fibroblasts | Adaption of mechanical properties to the lung tissue (alveolus) |
[86] | Matrigel, decellularized rat lung slices | Potential of AF-MSCs to generate lung precursor cells | In vitro | AF-MSC | AF-MSC are a possible cell source for cell therapy, differentiation on lung matrix more effective, limited amount of cell source, lack of cell proliferation |
[32] | Gelatin/microbubble-scaffolds | Vascularization of artificial scaffold for lung tissue engineering | In vitro, in vivo, in ovo, subcutaneous implantation in SCID mice | mPSC | Scaffolds promote angiogenesis and differentiation to alveolar pneumocytes |
The lung has a highly complex structure with varying structural composition and mechanical properties which are still unable to be completely recapitulated using synthetic approaches. While scaffolds fabricated via foaming techniques are structurally similar to peripheral lung tissue (especially the alveoli), they lack a vascular system and innervations. It is also difficult to tune the various mechanical properties needed throughout the lung for proper breathing motions. Additionally, the challenge of scaffold recellularization to create a fully functional organ has not yet been achieved. Thus, the use of the current methods exclusively may not solve the issue of whole lung replacement, but there are many areas for improvement which can still be explored.
Acellular Scaffolds
Synthetic scaffolds could one day be accurately and precisely manufactured for the macro- and microarchitecture required for ex vivo lung bioengineering. However, the instructional cues which are needed on the scaffold for critical events such as initial cell attachment, potential cell-specific attachment cues, and differentiation cues are not known. Furthermore, if these criteria were known, the lung scaffold would likely also need to be engineered with a material and manufacturing process selection which matched the mechanical and gas diffusion properties of native lung. This makes the engineering of a completely synthetic scaffold daunting. While synthetic materials could be engineered to include specific integrin binding sites to enhance cell adhesion (e.g., Arg-Gly-Asp (RGD) binding sites), it remains unknown what specific integrin binding sites need to be included and in what spatial arrangement they need to be. On the other hand, acellular scaffolds retain many of the native integrin binding sites in their correct spatial arrangement, and decellularization processes preserve the general organ architecture and ECM composition. Lung ECM has also long been known to provide instructional cues during prenatal development, postnatal tissue regeneration, remodeling responses following injury, and general tissue homeostasis [36–40]. Similarly, acellular scaffolds have been shown to have biologically inductive clues [21, 22, 27, 41, 42]. While hybrid materials, consisting of synthetic and acellular matrix components, are also an attractive possibility, these concepts are in their infancy. Hybrid materials could be utilized to enhance cell adhesion and biological activity while taking advantage of the ability to more precisely manufacture scaffolds or scaffold components with synthetic materials [21, 34, 35, 43, 44]. Differences between acellular and synthetic scaffold approaches are summarized in Table 18.2. Owing to the current advantages of acellular scaffolds, we will focus our discussion in the remainder of this chapter on their manufacture, assessment, and usage.
Table 18.2
Comparison of biologic vs. synthetic scaffold approach for ex vivo bioengineering
Biologic (acellular) scaffold | Synthetic scaffold | Potential hybrid design | ||||
---|---|---|---|---|---|---|
Differentiation and engraftment cues | + | Retains native integrin binding sites | − | Lacks specific integrin binding sites (must be engineered into scaffolds) | + | Could be engineered with specific ECM components or engineered integrin sites |
Immunogenicity | + | Antigen removal during decellularization | +/− | Unknown/variable depending on material | +/− | Unknown/variable depending on the artificial matrix material chosen |
Manufacturability | + | Native architecture largely retained | − | Complex architecture possible | − | Complex architecture possible |
− | Large variability between donor scaffolds | + | Precise control possible (i.e., repeatability) | + | More ability than acellular to be controlled, but ECM incorporation introduces a degree of variability | |
Long-term storage | − | Degradation with long-term storage | + | Improved storage stability | +/− | Improved storage stability, but would likely loose biologic activity under long-term storage |
Decellularization
Methods of Decellularization
The derivation of a cell-free ECM is not a new concept. Lwebuga-Mukasa and colleagues first described the generation of acellular lung scaffolds in 1986 for the study of rat type II alveolar epithelial (AEII) cell behavior on a native basement membrane [45]. This technique was heavily explored in simple tissues in the 1990s and early 2000s [46–49] and has made strides into the clinic. Acellular biologic scaffolds have been created from a variety of different simple tissues, including skin, esophagus, and trachea [4]. Decellularization was first applied to complex tissues using whole organ perfusion decellularization in heart in 2008 [15]. Beginning in 2010, several groups described similar techniques in lung [18–23] and since this time, the field has grown rapidly.
The basic goal of any decellularization technique is to remove the endogenous cell population while retaining the macroarchitecture of the organ or the tissue, along with the ECM composition (Fig. 18.2). Maintenance of mechanical tissue properties is also thought to be critical in evaluating decellularization protocols. A variety of methods have been described to decellularize tissue. Most commonly, a series or combination of detergents, solvents, acids/bases, and hypotonic or hypertonic solutions are used to remove the majority of cellular components. Alternative methods include physical methods such as freeze/thaw cycles and/or biological agents such as enzymatic treatment [48]. Methods of decellularization are comprehensively reviewed elsewhere [50]. In general, most protocols last from 1 to 7 days.
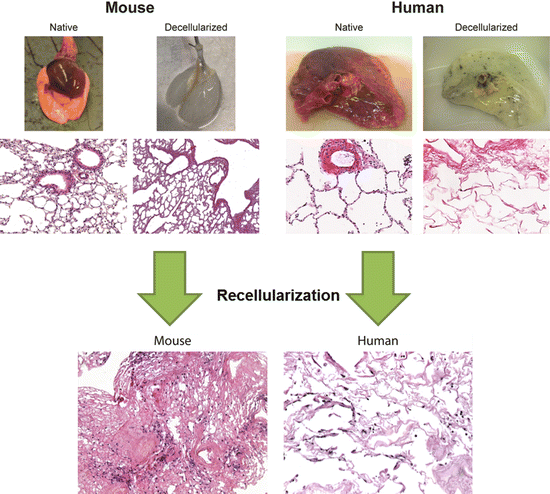
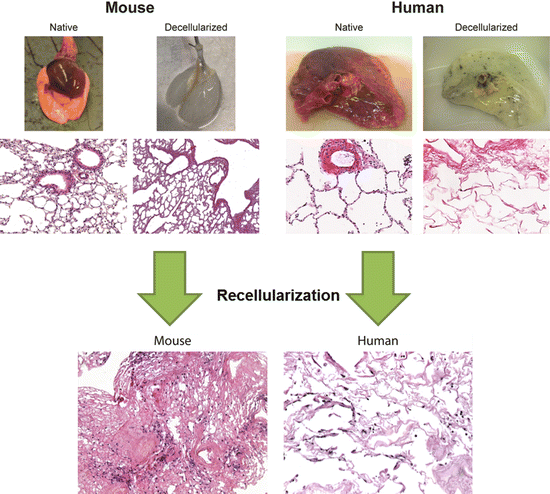
Fig. 18.2
Overview of the decellularization and recellularization process. Representative images of native and decellularized lungs from mice and humans (upper panel) demonstrating loss of pigmentation following decellularization, whereby the lungs become translucent white in color. H&E staining reveals complete cellular removal and gross maintenance of histological architecture. Histological analysis following recellularization with murine alveolar epithelial cells (C10) (left) and human bronchial epithelial cells (HBE) (right) into acellular mouse and human lung slices. Cells can be seen to have attached to the acellular lungs after 1 day of slice culture
There are a variety of published reports on techniques for decellularizing mouse, rat, porcine nonhuman primate and human lungs (Tables 18.3 and 18.4). In the lung, maintenance of both large and small airways and vessels is critical, in addition to the more delicate structures such as alveolar, capillary, and lymphatic systems which can be damaged through the use of excessive pressure during decellularization [51]. Perfusion decellularization has been most commonly utilized for whole lung decellularization, but there have also been reports of excising segments or slices from native lung and decellularizing these smaller segments [28, 29, 52, 53]. Detergents are the most commonly utilized decellularization agents used in perfusion based lung decellularization. There are several studies which have directly compared differences between these methods, and endpoint comparisons included assessment of proteomic composition, the mechanical properties of the final acellular scaffold, and recellularization efficacy [23, 41, 52]. The most commonly utilized detergents for lung are either the ionic detergents sodium deoxycholate (SDC) and sodium dodecyl sulfate (SDS), which are often used in combination with the nonionic detergent Triton X-100 [18, 22–27, 29, 41, 54–58]. Zwitterionic detergents such as 3-[(3-cholamidopropyl)dimethylammonio]-1-propanesulfonate (CHAPS) have also been used [19, 52, 59, 60], but some reports demonstrate that these may be more damaging than ionic or nonionic detergents due to their efficiency in denaturing proteins [48]. Many protocols also incorporate additional rinses and incubations for the purpose of removing organic components which are difficult to remove with the other detergents. The most commonly utilized additional steps are the use of hypertonic solution for lysis of cells (e.g., 1 M NaCl), or DNase/RNase to clear residual DNA and RNA. While both vascular-only perfusion and a combination of vascular and airway perfusion have produced acellular scaffolds capable of supporting recellularization, there is no consensus on the best route of administration and removal of decellularization agents.
Table 18.3
Compiled studies of ex vivo lung bioengineering using rodent and small primate decellularized whole lung scaffolds
Ref. | Scaffold | Study objective | Method of decellularization | Length of decellularization process | Endpoint assessments |
---|---|---|---|---|---|
[122] | Alveolar basement membrane (calf, dog, rabbit, adult/newborn rat) | Basement membrane | Filtered distal lung homogenate, saline, 4 % Triton X-100 with protease inhibitors, NaHCO3 rinse, distilled H2O rinse | 26–52 h depending on homogenate volume | Histology, IF, SEM, amino acid analysis, carbohydrate analysis |
[45] | Acellular alveolar vs. amniotic basement membranes | Differentiation on different basement membranes | Distilled H2O, 0.1 % Triton X-100, 2 % SDC, NaCl, pancreatic DNase Type 1S | >2 days | Cell attachment and morphology |
[20] | Mouse (female C57/BL6) acellular lungs | Effect of matrix on spatial engraftment of E17 fetal lung homogenate | Airway and vascular perfusion: distilled H2O, 0.1 % Triton X-100, 2 % SDC, 1 M NaCl, porcine pancreatic DNase | 3 days (approximately 63 h) | Histology, quantification of ECM proteins, IF, SEM, function with Flexivent, bioreactor with fetal type II cells |
[19] | Rat acellular lungs (male Fischer 344) | Development of bioartificial lung for orthotopic transplantation | Vascular perfusion only (1–5 mL/min with less than 20 mmHg arterial pressure) CHAPS, NaCl, EDTA, PBS | 4 h | Histology, IF, DNA quantification assay, collagen assay, GAG assay, western blots, SEM, TEM, micro-CT imaging |
[21] | Rat acellular lung (Sprague Dawley) | Comparison of matrices including decellularized rat lung in ability to support mESCs | Fast freeze/thaw cycles, 1 % SDS, DNase, RNase, PBS, Penicillin/Streptomycin, Amphotericin, DMEM | >6 weeks | Quantification of DNA, IH, confocal microscopy, flow cytometry, 2 photon microscopy, presence of SP-A |
[18] | Rat acellular lung (Sprague Dawley) | Development of bioartificial lung for orthotopic transplantation | Vascular perfusion only: pulmonary artery pressure kept constant at 80 cmH2O, heparinized PBS with 0.1 % SDS, deionized water, Triton X-100, and PBS with Penicillin, Streptomycin, Amphotericin B | 3 days (approximately 75 h) including incubation with antibiotics | Histology, morphology, mechanical function, fluoroscopy, gas exchange, transplantation, protein analysis |
[58] | Rat acellular lung (Sprague Dawley) | Orthotopic transplantation | Vascular perfusion only: pulmonary artery pressure kept constant at 80 cmH2O, heparinized PBS with 0.1 % SDS, deionized water, Triton X-100, and PBS with Penicillin, Streptomycin, Amphotericin B | 3 days (approximately 75 h) including incubation with antibiotics | Histology, IH, morphology, fluoroscopy, functional analysis, transplantation of seeded lungs with fetal pulmonary cells and Pulmonary artery and vein with endothelial cells |
[123] | Rat acellular liver and lung (Lewis) | Cellular differentiation on 3D in vitro scaffold | Lung lobes cut into 300 μm thick, 0.5 % Triton X-100, 10 mM ammonia, mechanical disruption, PBS, distilled water | N/A | Histology, TEM, environmental scanning, PCR, IH, liquid chromatography with tandem mass spectrometry |
[22] | Mouse acellular lung (C57BL/6; BALB/C) | Initial binding and recellularization of MSCs in acellular scaffold; directed seeding with integrin blocking | Same as [20] | 3 days (approximately 72 h) | Histology, IF, EM, dye perfusion to assess vascular continuity, mass spectrometry, western blot, lung mechanics with Flexivent, inoculation of bone-marrow-derived MSCs |
[23] | Mouse acellular lung and lung slices (BALB/C) | Comparison of detergent-based decellularization protocols | 3 days (approximately 72 h) | IH, mass spectrometry, western blot, mechanics with Flexivent, gelatinase assay, DNase, RNase, comparative recellularization with MSCs and C10s | |
[55] | Normal rhesus macaque acellular lung | Initial binding and recellularization of MSCs in acellular scaffold | Airway and vascular perfusion: PBS, EDTA, Penicillin/Streptomycin at initial harvest: pulmonary artery: PBS + Heparin + Sodium Nitroprusside with pressures 25–30 mmHg; then trachea and vasculature: deionized H2O [20] | 2–3 days (approximately 48–72 h) | Histology, morphology, IH, western blot, genomic DNA, proteomics, seeding with bone marrow and adipose derived rhesus MSCs |
[57] | Mouse acellular lung and lung slices (C57/BL6) | Seeding with and differentiation of mESCs-derived endodermal lung precursors | Same as [20] | 3 days (approximately 72 h) | Phenotypic assessment of mESCs and their capacity for differentiation |
[56] | Mouse acellular lung (C57BL/6) | Comparison of timing of decellularization, pre-coating, and support of mESCs and their differentiation capacity into alveolar epithelial cells | Same as [20] | 1 vs. 3 days (approximately 24 h vs. 50 h) | Histology, morphology, EM, western blot, gelatinase assay, IF, mechanical properties with Flexivent, viability of differentiated mESCs seeded into the scaffold, subcutaneous implantation of scaffold |
[60] | Rat acellular lung | Comparison of different detergent-based protocols | Two approaches: (1) [19]; (2) NaCl, EDTA, SDS | 4 h | Histology, collagen assay, elastin assay, GAG assay, DNA assay, mechanical testing with linear strips |
[99] | Rat acellular lung | Creation of perfusable human lung cancer nodules | Same as [18] | 3 days (approximately 75 h) including incubation with antibiotics | Recellularization with human A549, H460, or H1299 and cultured with perfused, oxygenated media for 7–14 days |
[54] | Mouse acellular lung and lung slices (C57BL/6) | Effect of time to necropsy, length of storage, and two different methods of sterilization | Same as [20] | 3 days (approximately 72 h) | Histology, IH, morphology, mass spectrometry, seeded lungs with MSCs and C10 epithelial cell line |
[24] | Mouse acellular lung and lung slices (C57BL/6) | Effect of recipient age and injury on de- and recellularization | Same as [20] | 3 days (approximately 72 h) | Histology, IH, mass spectrometry, inoculation with MSCs and C10 epithelial cell line |
[69] | Rat and mouse acellular lung slices | Engraftment and survival of fibroblasts through a β1-integrin and FAK-dependent pathway through ERK | Same as [19] | 1 Day | Histology, SEM, immunohistochemistry, DNA assay, recellularization with mouse A9 cells |
[41] | Rat, porcine, human acellular lungs | Evaluation of the capacity of acellular lung scaffolds to support recellularization with lung progenitors derived from human induced pluripotent stem cells (iPSCs) | Same as [18] | 1 Day | Histology, IF, quantitative PCR, implantation of rat acellular scaffold recellulalarized with iPSC, blood gas analysis in orthotopic transplantation |
[124] | Rat acellular lung | Comparison of different perfusions (manual, trachea + vasculature constant flow, vasculature only constant pressure) | Airway and vascular perfusion: PBS, 0.1 % Triton X-100, 2 % SDC, NaCl, Pancreatic DNase, MgSO4, CaCl2, PBS with Penicillin/Streptomycin and Amphotericin B | Up to 3 days | Histology, nucleic acid detection, IF, western blot, inoculation with C10 epithelial cell line |
[86] | Rat acellular lung | potential of AF-MSCs to generate lung precursor cells in the decellularized lung | Same as [124] | Approximately 1 day | Viability assay (TUNEL staining), IF, quantitative PCR |
[62] | Mouse acellular lung | Measurement of local stiffness by AFM | Same as [21] | >6 weeks | IH, IF, SEM, 2 photon microscopy AFM local stiffness measurement |
[125] | Rat acellular lung | Influence of pH during decellularization on ECM | Same as [19] | 4 + 48 h after treatment | Histology, IH, DNA quantification assay, collagen assay, GAG assay, western blot, TEM, subcutaneous implantation |
Table 18.4
Summary of decellularization methods for human and porcine lungs
Ref. | Species | Decellularization agents | Perfusion parameters | Instillation route | Days |
[19] | Human | CHAPS, NaCl, and EDTA | Constant pressure (25 mmHg) | Airway and vasculature | 1 |
[27] | Human/IPF | Triton X-100, SDC, NaCl, DNase | Unspecified | Airway and vasculature | 3 |
[126] | Human/porcine | SDS, Triton X-100 | Constant pressure (30 cmH2O) | Vascular | 4–7 |
[52] | Human/porcine | (a) SDS; (b) CHAPS; (c) Tween-20, SDC, peracetic acid | None—lung segments and agitation | N/A | 1 |
[44] | Human/porcine | Freeze/thaw; graded SDS perfusion | Varying flow rates (100–500 mL/h) | Airway and vasculature | 7 |
[61] | Porcine | Triton X-100, SDC, NaCl | 12–25 mL/min (15 mmHg) | Airway and vasculature | 1 |
[29] | Human/IPF | SDS, Triton X-100, NaCl | None—thin lung slices | N/A | 2 |
[26] | Human/porcine | Triton X-100, SDC, NaCl, DNase Peracetic Acid | Constant flow rates (1 L, 2 L, 3 L/min) | Airway and vasculature | 3 |
[25] | Human/COPD | Triton X-100, SDC, NaCl, DNase Peracetic Acid | Constant flow rate 2 L/min | Airway and vasculature | 3 |
[73] | Human/rat | SDS, Triton X-100 | Constant pressure (50 cmH2O) | Vascular | 4–7 |
[41] | Human/porcine/rat | (a) SDS; (b) SDC; (c) CHAPS | Constant pressure (30 cmH2O) | Vascular | 4–7 |
How differences in protocols and routes of administration for decellularization reagents might affect recellularization protocols or potential immunogenicity of implanted scaffolds is not yet known. There is currently no set of standards for demonstrating that a protocol has generated an optimal acellular scaffold. However, Crapo et al. proposed three minimal criteria: (1) <50 ng dsDNA per 1 mg ECM dry weight; (2) <200 bp DNA fragment length; (3) absence of visible nuclear content in histological sections by 4′,6-diamidino-2-phenylindole (DAPI) or hematoxylin-eosin (H&E) staining [50]. However, these are generic criteria for all acellular scaffolds and there are tissue and organ-specific requirements, such as preservation of mechanical properties that are likely important for lung. Furthermore, differences in retention of ECM components and mechanics have also been observed [23, 52] and these may be critical criteria in establishing lung-specific guidelines which must be met with the various protocols utilized in different laboratories.
Scaling Up Decellularization Protocols for the Clinic
Scaling up decellularization protocols from rodent lungs to potential clinical sources (e.g., large animal xenogeneic sources: e.g., porcine or human scaffolds) presents a new set of further challenges. In addition to anatomical differences, there are practical differences in handling organs of this size and it is not a simple matter of scaling up volumes. While rodent and macaque lungs have been decellularized by hand, higher pressures and volumes must be utilized for sufficient inflation of perfusion pathways (e.g., vasculature, airways, etc.) in larger organs. This ensures that perfused solutions reach distal airspaces and capillary beds and that the ensuing cellular debris is cleared from the lungs. All of the published protocols to date for decellularizing whole large animal or human lungs utilize perfusion pumps to generate acellular scaffolds which can support recellularization [19, 20, 25–27, 41, 44], and a recent report demonstrates a potential automated scheme which minimizes many of the practical issues [61]. While not a model for clinical translation, human and porcine lung segments have also been decellularized using small segments in order to improve high throughput study [28, 29, 52, 53] (Table 18.4). There are a variety of techniques which have been reported for assessing the efficacy of the decellularization protocol as well as for characterizing the remaining scaffold. Most reports characterize scaffolds using histologic, immunofluorescent staining, and DNA detection/quantification (Fig. 18.2). We will next discuss these endpoint assessments.
Residual Extracellular Matrix and Other Proteins
Owing to the importance of ECM components, retention of key ECM components is a critical parameter to assess as an endpoint when evaluating potential decellularization protocols. The precise combination of ECM proteins that must be retained to preserve the ability of the acellular scaffold to give organotypic cues for cellular differentiation and functional tissue level assembly remains unknown. The major structural and functional molecules in the ECM include proteins such as collagens, elastin, fibronectin, and laminins as well as a variety of glycoproteins including glycosaminoglycans (GAGs). Collagens are the chief structural components of the lung and are responsible for overall mechanical strength while elastin gives the lung its elastic properties of reversible distension and intrinsic recoil. GAGs help control macromolecular and cellular movement across the basal lamina and may also play a role in the mechanical integrity of the lung, although less is known about their exact role, matrix molecules are generally highly conserved proteins in eukaryotic organisms and therefore it is generally thought that these scaffolds will have minimal to no immune response if used in a xenogeneic context. This may theoretically explain the lack of an adverse immune response seen in xenotransplantation of other decellularized organs such as skin, trachea, and esophagus [4, 7, 11, 12].
There are a variety of techniques which have been used to evaluate ECM components, including histology, immunohistochemistry, western blotting, mass spectrometry-based proteomics, and component-specific assays such as Sircol Collagen Assay, Fastin elastase, etc. (Table 18.3). The majority of lung decellularization techniques result in significant loss of elastin and sulfated GAGs in all species studied thus far [18–20, 22–24, 54–56]. In head-to-head comparison studies of lung decellularization protocols, SDS and SDC have been found to retain more elastin as compared to CHAPS-based protocols [23, 60]. However, despite the differences in retention of ECM components, inoculated cells appear to behave similarly in the recellularization/repopulation assays currently used (including histological and immunofluorescence evaluation). Therefore, it remains unknown if there is an optimal decellularization protocol, and if so, which is best suited for translation to the clinic.
A recently emerging trend is the use of mass spectrometry proteomic analysis to help delineate differences between protein loss and retention in protocols or in scaffold source [22–26, 41]. This assessment has also been used to aid in the selection of optimal protocol parameters such as flow rates or pressures [26]. For example, proteomic analysis can help delineate the impact of changes in protocols during different steps, decellularization agents, or in decellularization parameters (e.g., flow rate, pressure, rinse volumes, etc.) by quantifying or semiquantitatively assessing which choices preferentially retain certain ECM components or minimize/maximize retention of cellular-associated proteins [25–27]. In addition to detecting ECM composition and residual proteins in acellular scaffolds, it has been used for distinguishing differences between decellularization methods or lung origin, including disease states or donor age [23–27, 41, 53, 55]. These assessments also yield critical information for those studying cell–ECM interactions as it can help delineate differences in the underlying matrix.
One particularly striking and consistent result amongst the various groups utilizing this analytical approach is the amount and breadth of non-ECM proteins detected in the scaffold following decellularization. In particular, cytoskeletal elements and cell-associated proteins appear to be retained in the scaffolds, while in general, lesser secreted proteins are detected. This suggests that transmembrane proteins and their associated cytoskeletal elements may remain anchored to the ECM with currently used decellularization protocols. The impact of these residual proteins on recellularization, including potential immunogenicity remains unknown. Furthermore, in the current reports, proteomic assessment has been limited in scope and generally only the most abundantly expressed proteins are reported.
Mechanical Assessments of Decellularized Scaffolds
A variety of in vitro assessments have been utilized to assess the mechanical properties of acellular scaffolds. Investigators have explored both micro-[62, 63] and macroscale [22, 23, 44, 64, 65] mechanical measurements of acellular lungs as well as force tension relationships in linear strips of decellularized lungs [52, 60]. While techniques such as atomic force microscopy (AFM) are useful in obtaining topographical information and initially assessing mechanical properties of the scaffolds [27, 62, 63, 65], these results have yet to be correlated to recellularization or functional performance. Traditional lung mechanics testing of acellular scaffolds has shown that in the absence of cells and surfactants, acellular scaffolds are stiffer than their naïve counterparts [22]. Introduction of exogenous surfactant into the acellular scaffolds can partially restore lung compliance [22]. This is an important finding and indicates that during recellularization strategies, serial measurements of lung mechanics could be used as a noninvasive and nondestructive means to assess functionality of the regenerating scaffold. For example, decreases in elastance could be used as a measurement of de novo surfactant production. However, as acellular lungs are often leaky following decellularization, interpreting results in this context can be challenging [44]. The importance and challenges of measuring mechanical properties in ex vivo bioengineering is discussed in more detail in the review by Suki [66].
Recellularization
Recellularization of Acellular Scaffolds for Bioengineering New Lung
The lung is a complex organ with a variety of different functions. These include gas exchange, immune system surveillance, and ciliary clearance of inhaled foreign objects. In order to accomplish all of these diverse functions, lung tissue utilizes a variety of different cell types, all of which uniquely contribute to some critical aspect of lung function [67]. Following a variety of acute injuries, such as infection or chemical insult, the lung has the capability to repair itself through activation of endogenous regeneration. The heterogeneous cell population of the lung is replenished by resident stem or progenitor cells, which differentiate into the various adult cell types [68]. Once implanted, it is thought that any ex vivo regeneration strategy must recapitulate these functions, whether it is through a completely biological strategy (i.e., functioning tissue) or some combined artificial and biological solution. It is therefore likely that lung tissue grown ex vivo require some minimal restoration of these subtypes so that it will function once transplanted.
While a variety of cell sources are being investigated for recellularizing acellular and artificial scaffolds, obtaining sufficient cell numbers with any source remains a significant open question. The ideal solution is thought to be the usage of an autologously derived source of cells to minimize post-transplantation immune complications which are a significant cause of morbidity in transplanted patients. One potential source is the use of fully differentiated primary adult cells. However, these cells may not have sufficient replicative capacity to fully recellularize the organ, plus, normal repair and regeneration following normal lung injury (e.g., illness) may not be possible. Nevertheless, these sorts of repopulation studies may shed light on recellularization strategies using other cell types. It remains unknown if multiple cell types could be isolated from the eventual transplant recipient, grown to sufficient numbers ex vivo and then used in a recellularization approach to restore functionality. While it has been shown that a strategy such as integrin blocking can be used to direct initial cell engraftment of a single cell population [22, 69], scaling this clinically and further adding the complex challenge of uniquely directing the right cell population to a specific architectural location would be challenging. Alternatively, autologous endogenous lung progenitor cells from the various compartments could be utilized (e.g., distal and proximal epithelial progenitor cells, endothelial progenitor cells, etc.) along with stromal cells to recellularize acellular scaffolds. However, the same challenges of obtaining sufficient cell numbers for an initial seeding strategy and directing cells to their correct compartment remain. In both instances, it remains unknown if normal cells could be obtained from a patient with a preexisting lung disease or if isolated diseased cells could be gene-corrected prior to subsequent recellularization. Recent work indicates that the scaffold may more significantly contribute to phenotype than cell-origin. Fibrotic scaffolds were found to induce a pro-fibrotic profile, independent of whether normal or IPF-derived human fibroblasts were used in repopulation assays, whereas the normal lung scaffold did not induce a pro-fibrotic profile if either cell type was used [29]. An allogeneic cell source could also be used, but this re-introduces the potential for immune complications following transplantation. Furthermore, the identification of bona fide distal airway lung progenitor cells in the adult human lung remains controversial.
A potentially more appealing autologous approach is the use of induced pluripotent stem cells (iPS) which are derived from reprogramming somatic cells to a stem-cell-like state. While iPS cells avoid the ethical controversies surrounding the use of embryonic stem cells (ESCs)—stem cells derived from the inner blastocyst of in vitro fertilized embryos—iPS cells have been shown to retain epigenetic memory of their tissue origin and have been shown to form teratomas [70]. iPS cells are typically derived from dermal fibroblasts and thus, differentiating them into the various lung cell types has been challenging. However, despite this limitation, recent work has demonstrated that human iPS cells can be differentiated into cells expressing a distal pulmonary epithelial cell immunophenotype and seeded into acellular human lung scaffolds [71–73]. These results further encourage the use of this approach in moving towards the clinic.
Other potential approaches include the use of fetal homogenates or ESCs. As previously mentioned, ethical concerns remain for either of these approaches, as well as the potential for teratoma formation with ESCs. While initial studies have shown that ESCs can engraft in acellular murine lungs [21, 57], seeding into acellular lungs was not sufficient to induce differentiation. Optimized in vitro differentiation protocols must be used in conjunction with seeding and repopulation strategies. Significantly, ESC-derived murine Nkx2-1GFP+ progenitor cells were able to recellularize acellular murine lungs and form alveolar structures, while in contrast, seeding with undifferentiated ESCs resulted in nonspecific cell masses in distal regions of acellular lungs. Fetal homogenates have the distinct advantage of containing all the necessary cell populations, and have been shown to have some capacity for self-assembly. These cells have been successfully used in the current rodent models of ex vivo regeneration and transplantation. However, in both instances, ethical concerns remain in obtaining these cells and the need for immunosuppressive drug treatment post-transplantation remains unknown. Tables 18.5 and 18.6 summarize recellularization approaches in animal and human models and the phenotype adopted by seeded cells.
Table 18.5
Distribution and phenotype of cells seeded onto animal models of acellular scaffolds
Ref. | Cells used for seeding | Scaffold | Route | Duration | Distribution | Final phenotype |
---|---|---|---|---|---|---|
[45] | ATII | Acellular alveolar vs. amniotic basement membranes
![]() Stay updated, free articles. Join our Telegram channel![]() Full access? Get Clinical Tree![]() ![]() ![]() |