Fig. 15.1
Circulating and resident cells involved in neoangiogenesis. This figure depicts the rare circulating low proliferative potential (LPP) and high proliferative potential endothelial colony forming cells (HPP-ECFC) that may become the new vessels at neoangiogenesis sites. More abundant circulating hematopoietic cell subsets modulate the initiation, recruitment, and formation of the new vessels via stimulation of the circulating and resident ECFC
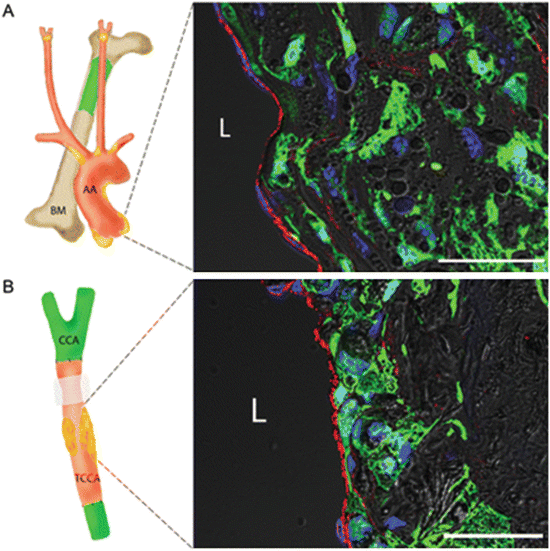
Fig. 15.2
Plaque ECs were derived from the local arterial wall. (a) Experiment to investigate whether or not bone marrow (BM)-derived endothelial progenitor cells (EPCs) contribute to plaque ECs during atherogenesis. An aortic root plaque from an apoE−/− mouse transplanted with BM from an eGFP+apoE−/− mouse. No eGFP+vWF+ double-positive cells are present. (b) An experiment to investigate whether or not any types of blood-borne EPCs contribute to plaque ECs during atherogenesis. A common carotid artery (CCA) segment from an apoE−/− mouse was orthotopically transplanted into eGFP+apoE−/− mice (isotransplantation except for the eGFP transgene). None of the vWF+ cells is eGFP+. Green indicates eGFP; red vWF; blue nuclei; gray DIC. L lumen; BM bone marrow; AA aortic arch; CCA common carotid artery; TCCA transplanted common carotid artery. Scale bars = 50 μm. Reproduced with permission from Hagensen et al. [39]
In contrast, it has been known for more than 50 years that rare viable circulating endothelial cells (CECs) are present in the blood stream of goats, baboons, dogs, pigs, and human subjects and that these CECs can function to form an intraluminal endothelial lining covering implanted Dacron materials or intravascular devices [41]. Of interest, the circulating blood cells in young pigs also contain fibroblast and smooth muscle cells that form an adventitial subendothelial network on Dacron materials suspended in the flowing blood in the thoracic aorta. A variety of factors appear to play important roles in determining whether a large implanted device will develop an intact coating of endothelial cells and these include, (1) host species, (2) age of host, (3) device surface composition, and (4) dimensions of the device (reviewed in [42]).
The CEC present in adult peripheral and umbilical cord blood display heterogeneity in clonal proliferative potential [43, 44]. Given the ability of the CEC to generate clonal colonies of endothelial cells, these cells have been called endothelial colony forming cells (ECFC) (Fig. 15.3). Cord blood and adult blood ECFC display similar cell surface antigen expression of proteins common to all endothelial cells, fail to express blood markers such as CD45 or CD14, and form human blood vessels that integrate with the host circulation upon transplantation into immunodeficient mice [30, 45–49]. Differences in expression of telomerase and maintenance of telomeres exists between cord blood and adult peripheral blood ECFC, consistent with significantly greater population doublings achieved by cultured cord blood ECFC before reaching replicative senescence. No unique identifying marker has yet been found to identify the ECFC within the human blood cells to permit prospective isolation, though all of the viable ECFC present in cord blood are present within a subset of cells with the phenotype CD34+CD31+CD146+CD45−CD133− [50]. It is unclear what role the circulating ECFC play within the human vasculature, however, recent studies implicate a role for the in vivo selectin-mediated adhesion of circulating human ECFC at the site of thrombus stabilization via recruited neutrophils. This neutrophil and ECFC interaction activates the proangiogenic functions of the ECFC and may be involved in vessel repair [51]. The number of circulating ECFC has been shown to highly correlate with the severity of coronary artery stenosis in symptomatic patients after adjusting for age, gender, cardiac risk factors, left ventricular ejection fraction, angiotensin-converting enzyme inhibitor or statin use [52]. The presence of higher concentrations of circulating ECFC is associated with reduced microvascular obstruction after acute myocardial infarction, leading to reduced infarct size and less left ventricular remodeling in patients compared to those patients in whom circulating ECFC could not be isolated [53]. The circulating concentration of ECFC has also been documented to increase tenfold following an acute myocardial infarction in human subjects [54], but a comparison of patient outcomes based upon the extent of the acute mobilization of these cells during myocardial infarction has not yet been conducted. These data all suggest a relationship between the presence of myocardial ischemic protection and circulating ECFC concentrations in human subjects. Of interest, the source of the circulating ECFC remains unknown.
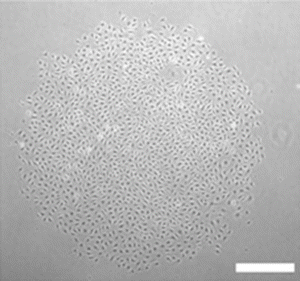
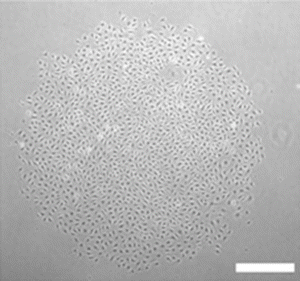
Fig. 15.3
Representative photomicrograph of endothelial colony. Colony formed 7 days after adult and cord blood-derived endothelial cells were plated at low cell density (×50 magnification). Scale bar represents 100 μm
Whereas circulating apoptotic and necrotic endothelial cells have long been thought to be sloughed vascular endothelial cells that increase in most cardiovascular diseases and cancer [55, 56], the specific site and mechanisms for generating viable ECFC remain obscure [42]. Duong et al. [57] have reported that directly plating of pulmonary artery endothelial cells (PAEC) isolated from vessels from patients with pulmonary hypertension revealed significantly greater number of clonogenic ECFC from the patient samples than from control subject lung vessels. They concluded that patient-derived PAEC contain significantly more ECFC that display greater proliferative potential than control subject PAEC. We have also identified ECFC resident in the endothelium isolated from umbilical cord and aortic endothelium of human subjects [58] that display high proliferative clonogenic potential. In human subjects with peripheral arterial disease, circulating ECFC display diminished proliferative potential that is matched by diminished ECFC numbers and proliferative potential by resident arterial samples [59]. Lin et al. [44] identified the most proliferative circulating ECFC to arise from the donor bone marrow tissue in patients that had undergone a sex-mismatched bone marrow transplant. Whether the ECFC were derived from vessels contained in the marrow tissue or from the hematopoietic cells that reconstituted the host subjects has not been clarified.
In sum, the strength of evidence supports an important role for resident local vascular endothelial cells to participate in repair of experimentally and genetic-induced endothelial injury or disruption in rodent models and that process is facilitated via paracrine molecules provided by circulating proangiogenic hematopoietic cells (previously called endothelial progenitor cells) recruited from the bone marrow. There is limited evidence for circulating endothelial cell contribution to vascular injury models in mice or rats, however, in larger mammalian species, substantial evidence implicates the presence of viable circulating endothelial cells that can cover intravascular implanted devices and circulating ECFC play a role in protection of the cardiac microcirculation following an acute infarction that promotes better patient outcomes.
Vascular Regeneration in Post-pneumonectomy Lung Growth
It has been recognized for more than a century that surgical removal of lung tissue in numerous animal species is associated with compensatory lung growth by the remaining lung tissue (reviewed in [60]). While compensatory growth was successfully demonstrated in dogs and rabbits in 1881, attempts to successfully remove lung tissue in human subjects failed to lead to successful outcomes until the 1930s (reviewed in [61]). Whether or not the human lung responds to lung resection with compensatory growth appears to be related to many factors including age. Several reports have documented long-term increases in lung volume (without an increase in residual volume) indicative of new alveolar growth following pneumonectomy [62–64]. The debate over whether the human adult lung can undergo compensatory growth following pneumonectomy remains controversial (reviewed in [60]). Continued advances in studying the mechanisms leading to compensatory growth in animal model systems have provided important understanding for how the host responds to loss of lung tissue with changes in cardiac and respiratory physiology, while advances in respiratory support and numerous surgical tools and approaches have steadily improved outcomes in this challenging operation.
Many physiologic factors are known to regulate the compensatory growth of the lung to pneumonectomy in animal model systems. These variables include the species, sex, and age of the animal tested [65, 66]. The impact of stretch on the remaining lung is known to be a major factor in the extent of compensatory lung growth observed post-pneumonectomy [67, 68]. Since the entire cardiac output is diverted into the remaining lung post-pneumonectomy, significant changes in pulmonary blood flow also impact the compensatory growth response [69, 70]. The specific roles played by the numerous cell types that comprise the lung in the realveolarization process that exemplifies compensatory lung growth have only recently begun to be elucidated.
Since sprouting angiogenesis is a prominent feature of the pulmonary capillary bed during normal lung development [71–73] and there is a parallel increase in the number of endothelial cells and the nearly 30 % increase in alveoli post-pneumonectomy [74], numerous authors have considered that angiogenesis may play a prominent role in post-pneumonectomy lung growth [75–77]. It is well known that a host of angiogenic growth factors are elevated in the lungs of animals post-pneumonectomy [78–82]. In fact, there are several waves of changes in gene expression that occur in the capillary bed post-pneumonectomy that may drive the angiogenic response [83].
Ding et al. [84] have provided recent analysis of some of the cellular and molecular mechanisms involved in compensatory lung growth following pneumonectomy in mice. Within 2 weeks of removal of the left lung, a 1.5-fold increase in right lung weight and 1.8-fold increase in right lung volume was observed. A significant increase in proliferation was documented in Clara cell secreted protein (CCSP) expressing bronchoalveolar stem cells (BASC) as early as day 3 post-pneumonectomy prior to any change in proliferation of type II alveolar epithelial cells (AECIIs) or pulmonary capillary endothelial cells (PCECs). However, proliferation in the AECIIs and PCECs is significantly increased by day 7, such that the proliferating PCECs constitute 7 % of the total lung mononuclear cells. By day 15 post-pneumonectomy, both AECIIs, which are known to be precursors of the type 1 AEC, and PCECs are increased threefold over sham control populations. Since growth activated endothelial cells are known to secrete a variety of angiocrine factors that can promote tissue repair and regeneration [85, 86], Ding et al. examined whether PCEC displayed evidence of VEGFR2 or fibroblast growth factor receptor 1 (FGFR1) activation. Though the overall level of VEGFR2 was not increased in PCEC, VEGFR2 phosphorylation was significantly increased on days 1–9 post-pneumonectomy, while FGFR1 was increased on days 3–11 post-pneumonectomy. These results implicated activation of these growth factor receptors in the PCEC as a potential key element in the PCEC expansion that occurred during lung regrowth. When VEGFR2 and/or FGFR1 were subsequently deleted specifically in endothelial cells in transgenic mice, left lung pneumonectomy failed to increase proliferation in the BASC population of the right lung at day 3 post-pneumonectomy, completely abrogated proliferation in the AECII and PCEC in the remaining lung at day 7 and beyond, and severely diminished restoration of lung weight and total lung volume in the right lung post-pneumonectomy. These results suggested that the growth factor-stimulated PCEC may be playing a significant role in the realveolarization of the lung post-pneumonectomy. In a series of additional studies the authors provided evidence that the PCEC of the regenerating right lung secreted high concentrations of matrix metalloproteinase 14 (MMP14), that PCEC MMP14 enhanced proliferation of AECII cells and BASC in a cell–cell contact interaction, and that inhibition of PCEC MMP14 with a blocking antibody in vivo blocked alveolarization in the remaining lung post-pneumonectomy. The specific mechanism through which MMP14 stimulated alveolarization appeared to be via unmasking of cryptic epidermal growth factor (EGF)-like ligands (from cleaved laminin matrix molecules) that stimulated the epithelial regrowth via the EGF receptor. Indeed, intravenous infusion of recombinant EGF restored alveologenesis post-pneumonectomy even in transgenic mice lacking both VEGFR2 alleles and one FGFR1 allele in PCEC. Of interest, intravenous infusion of lung PCEC, but not liver sinusoidal endothelial cells, from wild-type littermate controls also rescued alveologenesis and lung regrowth post-pneumonectomy in transgenic mice lacking PCEC expression of VEGFR2 and FGFR1. Ding et al. concluded that pneumonectomy induces a pulmonary specific activation of PCEC in the remaining lung that results in release of specific angiocrine factors that promote alveologenesis from BASC and AECII precursors and this collective endothelial–epithelial interaction leads to compensatory lung growth in adult mice (Fig. 15.4).
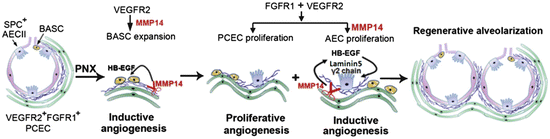
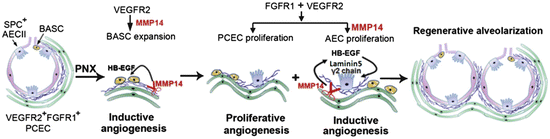
Fig. 15.4
Proposed model illustrating the inductive role of VEGFR2 and FGFR1 primed PCECs in lung regenerative alveolarization. Upon left pneumonectomy, activation of VEGFR2 in PCECs leads to MMP14 production and HB-EGF release to stimulate the expansion of epithelial progenitor cells (BASCs and AECIIs). Subsequent activation of FGFR1 along with VEGFR2 stimulates proliferation of PCECs maintaining MMP14 expression. MMP14 unmasks cryptic EGFR ligands through shedding of HB-EGF and cleaving laminin5 γ2 chain, which by activating EGFR induce proliferation of SPC + E-cadherin + AECs. After pneumonectomy, sequential propagation of epithelial cells induced by PCEC-derived MMP14 and increase in bioavailability of EGFR ligands, culminates in full reconstitution of physiologically functional alveolar-capillary sacs. Proliferation of the PCECs mediated through VEGFR2 and FGFR1, vascularizes the regenerating lung tissue to restore the blood supply and gas exchange function. Abbreviations: VEGFR2 vascular endothelial growth factor receptor 2; FGFR1 fibroblast growth factor receptor 1; PCEC pulmonary capillary endothelial cells; MMP14 matrix metalloproteinase 14; HB-EGF heparin binding epidermal growth factor; BASCs basal alveolar stem cells; AECIIs alveolar epithelial type II cells; EGFR epidermal growth factor receptor; SPC surfactant protein C; E-cadherin epithelial-cadherin. Reproduced with permission from Ding et al. [84]
Other investigators have focused on understanding the different patterns of neovascularization observed in the remaining lung following pneumonectomy. New blood vessel growth can occur via sprouting angiogenesis from preexisting vessels or by non-sprouting intussusceptive angiogenesis [87]. During intussusceptive vessel remodeling, the opposing walls of the vessel are bridged together and become sealed as a pillar that now separates the vessel into two vessels and as the new vessels mature, small holes 1–5 μm in diameter appear in between the vessels where the pillars originally formed [88–90] (Fig. 15.5). A detailed analysis of post-pneumonectomy lung growth by scanning electron microscopy, microCT scans, synchrotron radiation tomographic microscopy, and light and transmission electron microscopy has revealed that there is asymmetric growth of the right lung when the left lung is removed in rodents with the right cardiac lobe displaying the greatest expansion into the left pleural space [75, 91]. Surprisingly, many of the features of normal lung development are observed in the regenerating lung with upfolding of new alveolar septa and a duplicated capillary bed. This duplication is caused by massive intussusceptive angiogenesis and appears to be critical for the septal alveolarization in the regenerating lung just as it is in normal lung development [91] (Fig. 15.6). These data strongly implicate the resident capillary endothelial cells as the primary agents driving the vascular reparative response. However, the capillary endothelial cells do not function in a vacuum, as there are robust increases in recruited CD11b+ myeloid cells, alveolar macrophages, and AECII in close apposition to the pulmonary capillary endothelial cells and these recruited cells display an increase in angiogenic growth factor secretion during this phase of angiogenesis; disruption of the recruitment of these accessory cells diminishes the angiogenic response and the extent of lung regeneration indicating an important role for these diverse cell types [91–93].
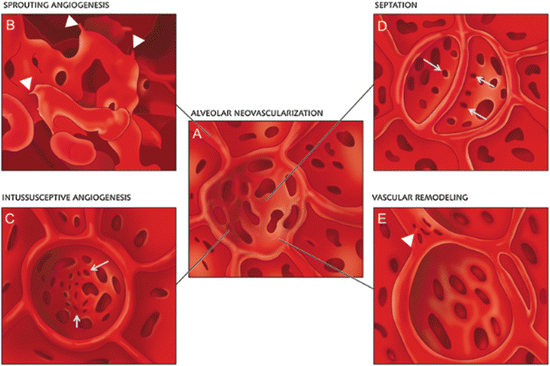
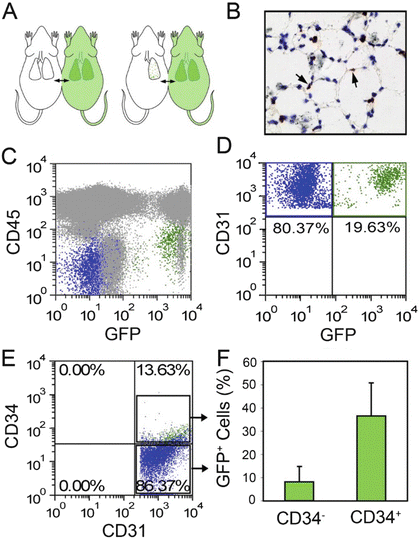
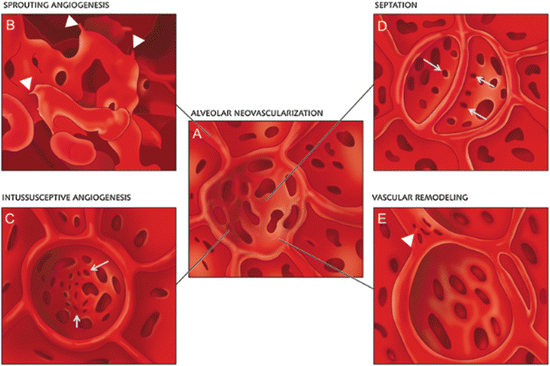
Fig. 15.5
Schematic illustration of alveolar neovascularization (a) as seen in microvascular corrosion cast replicas with sprouting angiogenesis (b), which is frequently seen subpleurally and in compact growth zones. Sprouts are evident as blind ends or protrusions (arrowheads). (c) Alveolar intussusceptive angiogenesis, recognizable by the presence of numerous small caliber holes with diameters between 1 and 5 μm as hallmarks of pillar formation (arrows). (d) The formation of new alveolar septa is accompanied by the occurrence of parallely orientated intussusceptive pillars (arrows)—visible in the cast as holes—ensuring a rapid expansion of the alveolar microvascular network. Vascular remodeling also occurs on the AER vessels (arrowheads). Intussusceptive pillars may merge and split up the primary vessel. Reproduced with permission from Ackermann et al. [91]
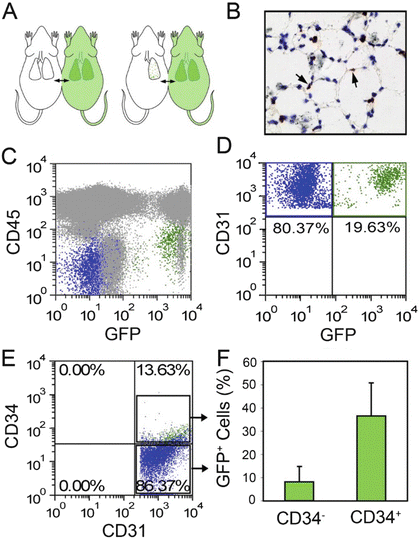
Fig. 15.6
Parabiotic demonstration of blood-borne CD34+ endothelial cells on day 7 after pneumonectomy. (a) Schematic of experimental design involving a left pneumonectomy in the wild-type parabiont of wild-type/GFP+ parabiotic twins (C57/B6). Parabiosis was established for 28 days prior to left pneumonectomy. The remaining lung was studied 7 days after pneumonectomy. (b) Anti-GFP avidin–biotin complex (ABC) immunohistochemical staining demonstrated GFP+ cells within the alveolar septae. Flow cytometry of the lung digests demonstrated GFP+ cells that were CD45− (c) and CD31+ (d). (e, f) Analysis of CD31+ lung endothelial cells demonstrated that most of the GFP expression occurred in the CD34+ endothelial cell population. Reproduced with permission from Chamoto et al. [92]
Chamoto et al. [92, 94] have recently proposed that blood-borne CD34+ endothelial progenitor cells displaying enhanced proliferative status are recruited into the pulmonary circulation post-pneumonectomy and become resident endothelial cells during the period of compensatory lung growth. Whereas only 1 % of lung endothelial cells expressed CD34 at homeostasis, a dramatic 12-fold increase in CD34+ lung endothelial cells was detected at day 7 post-pneumonectomy and the frequency of CD34+ endothelial cells returned to nearly normal levels by day 21 following lung resection. At day 7 post-pneumonectomy, cell division was fourfold greater in the CD34+ lung endothelial cells than in the CD34− lung endothelial cells. In an attempt to interrogate the contribution of blood-borne CD34+ cells to the CD34+ lung endothelial pool post-pneumonectomy, the authors surgically connected a wild-type mouse with a transgenic constitutive green fluorescence protein (GFP) expressing mouse via parabiosis [92, 95]. Following a 28-day recovery period, the wild-type parabiont underwent left pneumonectomy and 7 days later the regenerating right lung was analyzed for evidence of GFP+ blood-borne cells (Fig. 15.6). Though poorly visualized by fluorescence microscopy, GFP+ cells were detectable by immunohistochemistry in the alveolar septa. Approximately 11 % (range 4–20 %) of the CD31+ lung endothelial cells were GFP+ and more than 1/3 of the CD34+ endothelial cells in the lung were GFP+ (Fig. 15.6). Intravenous infusion of several different fluorochrome labeled lectin molecules prior to sacrifice of the animals and digestion of the lung tissue, demonstrated uniform labeling of the CD34+ and the CD34− lung endothelial cells, suggesting integration of the CD34+ cells into the endothelial intima. These and other data led the authors to conclude that blood-borne bone marrow-derived CD34+ EPCs were recruited and integrated into the lung circulation during the post-pneumonectomy lung growth. While the use of flow cytometry enhanced the ability of the authors to detect the presence of the dim GFP+ cells within the lung endothelium, this tool lacks the ability to provide anatomic localization of the cells within the vasculature. Ohle et al. [96] have previously reported that confocal microscopy analysis of putative bone marrow-derived GFP+ EPC contributions to injured lung has revealed that in essentially all events examined, the flat GFP+ cells near a vessel lumen were located in a periendothelial location and were not bona fide GFP+ endothelial cells. Since Chamoto et al. [92] showed that CD34+ expression increases in the lung post-pneumonectomy independent of recruited GFP+ cells (Fig. 15.6e), it is possible that some of the recruited CD34+GFP+ cells from the GFP+ parabiont may have been derived as mobilized lung endothelial cells. A direct analysis of the cells circulating in the blood of the parabionts at rest and following pneumonectomy would be required to test this hypothesis. At present the majority of published work (above) suggests that the primary endothelial cells that participate in the angiogenic response that drives neoalveolarization and lung regeneration post-pneumonectomy arise from lung resident endothelial cells.
Vascular Repair and Regeneration in Lung Injury
Abounding evidence suggest the supportive role of circulating proangiogenic hematopoietic cells in the repair of acute and chronic lung injury. In the classic model of murine acute inflammatory lung injury induced by lipopolysaccharide (LPS), there was a rapid release of putative EPCs into the circulation that contribute in concert with other bone marrow-derived progenitor cells to lung repair [97]. Transplantation of autologous putative EPCs as a therapeutic strategy attenuated endotoxin-induced lung injury in rabbits [98]. Huang and Zhao [99] elegantly demonstrated the importance of the resident local vascular endothelial cells and their interaction with circulating bone-marrow-derived progenitor cells (BMPC) to insure lung repair by using a mouse model with endothelial cell-restricted disruption of FoxM1 (FoxM1 CKO) [100]. In wild-type mice, BMPC treatment improved LPS-induced lung inflammation and survival and lead to a rapid induction of FoxM1 expression. Conversely, these effects were abrogated in FoxM1 CKO mice and BMPC treatment failed to induce lung EC proliferation suggesting that endothelial expression of the reparative transcriptional factor FoxM1 is required for the protective effects of BMPCs. Human studies also suggest a supportive reparative role of circulating putative EPCs in acute and chronic lung diseases. Patients with acute lung injury have twofold higher numbers of circulating putative EPCs than healthy control subjects, suggesting some biological role for the mobilization of these cells during lung disease [101]. Interestingly and similar to the prognostic role of EPCs in ischemic vascular diseases, improved patient survival in acute lung injury correlates with increased circulating putative EPCs [102] and severity of illness [101]. Likewise, the number of circulating putative EPCs is significantly increased in patients with pneumonia and patients with low EPC counts tend to have persistent fibrotic changes in their lungs even after recovery from pneumonia. Likewise, the number of circulating putative EPCs is significantly increased in patients with pneumonia and patients with low EPC counts tend to have persistent fibrotic changes in their lungs even after recovery from pneumonia [103]. Decreased numbers and impaired mobilization of circulating putative EPCs have also been reported in patients with pulmonary fibrosis and chronic obstructive pulmonary disease (COPD), but remain controversial [103–105]. More interesting is the study of the function of these cells. Blood outgrowth endothelial cells (BOECs; also called ECFC) from smokers and COPD patients showed increased DNA damage and senescence as well as impaired angiogenic ability compared to nonsmokers [106]. BOECs senescence could be reversed by activating the protein deacetylase SIRT1. In summary, these data largely confirm the supportive role of circulating proangiogenic hematopoietic cells in the pathophysiology and repair of acute and chronic lung injury (though in many cases it is unclear exactly which circulating blood cell subset was comprising the EPC population evaluated).
Additional evidence for the important role of lung resident endothelial cells in lung repair comes from the literature in the developing lung. Bronchopulmonary dysplasia (BPD), the chronic lung disease that follows ventilator and oxygen therapy for acute respiratory failure after premature birth, now predominantly occurs in infants born <28 weeks gestation, is characterized by an arrest in alveolar development and decreased lung vascular growth. Borghesi et al. [107] studied ECFC in the cord blood of 98 preterm infants (gestational age <32 weeks). ECFCs in cord blood were lower in infants who later developed BPD and even though ECFCs decreased with decreasing gestational age, extremely low gestational age infants (gestational age <28 weeks) with higher numbers of cord blood ECFCs were protected from BPD. The endothelial and hematopoietic cell subsets studied by flow cytometry were comparable in infants with and without BPD and rapidly decreased after birth. Conversely, the same group showed that the percentages of circulating angiogenic cells (CD34+VEGFR-2+; CD34+CD133+VEGFR-2+; and CD45−CD34+CD133+VEGFR-2+ cells) in the peripheral blood at birth, day 7 and day 28 of life in 142 preterm neonates (gestational age <32 weeks) were not able to predict the development of BPD [107]. Baker et al. quantified ECFCs and the angiogenic circulating progenitor cells (CPC)/nonangiogenic-CPC ratio (CPC/non-CPC) in cord blood samples from 62 preterm infants (24–36 weeks gestational age) [108]. ECFC number and CPC/non-CPC ratio were significantly decreased in cord blood of preterm infants who subsequently developed moderate or severe BPD. These data suggest that decreased circulating ECFCs in the fetal circulation impairs postnatal vasculogenesis thereby contributing to the severity of chronic lung disease in preterm infants.
Histological abnormalities of BPD are recapitulated in neonatal rodents exposed to chronic hyperoxia [109]. This model exhibits decreased circulating, lung and bone-marrow-derived putative EPCs [110]. Interestingly, hyperoxic adult mice did not display alveolar damage and had increased circulating putative EPCs, implying that decreased EPCs may contribute to the arrested lung growth seen in the neonatal animals. Infusion of these bone marrow-derived angiogenic cells restored alveolar and lung vascular growth in neonatal mice exposed to hyperoxia [111]. The recent discovery in the adult rat lung of resident microvascular endothelial progenitor cells that share features of human cord blood and lung ECFCs provided an opportunity to explore the role of resident ECFCs in experimental BPD models. Alphonse et al. [112] were able to show that the developing human fetal and neonatal rat lungs contained ECFCs with robust proliferative potential, secondary colony formation, and de novo blood vessel formation in vivo when transplanted into a matrigel plug under the skin of immunodeficient mice [112]. In contrast, human fetal lung ECFCs exposed to hyperoxia in vitro and neonatal rat ECFCs isolated from hyperoxic alveolar growth-arrested rat lungs mimicking BPD proliferated less, showed decreased clonogenic capacity, and formed fewer capillary-like networks. This suggested that impaired ECFC function may contribute to explain the incapacity of lung repair and the persistent alveolar growth in BPD. It also provided the rationale to test the therapeutic potential of exogenous supplementation of human cord blood-derived ECFCs. Because of the increased toxicity of hyperoxia on ECFCs [113, 114], intrajugular administration of human cord blood-derived ECFCs was performed after established oxygen-induced arrested alveolar growth. ECFCs restored lung function, alveolar and lung vascular growth, and attenuated pulmonary hypertension in immune-deficient rats and mice. Lung ECFC colony- and capillary-like network-forming capabilities were also restored. The therapeutic benefit persisted at 10 months of age with no adverse effects and persistent improvement in lung structure, exercise capacity, and pulmonary hypertension. However, lung engraftment, similar to what has been observed with other types of cell therapies (mesenchymal stromal cells for example) was low, suggesting that the effect of these exogenous ECFCs was through a paracrine effect. Accordingly, administration of cell-free ECFC-derived conditioned media exerted similar therapeutic benefits in the hyperoxia- and bleomycin-induced models of BPD in rodents [112, 115]. Overall, these findings further support the crucial role of lung resident endothelial cells in lung repair and offers promising new therapeutic strategies to prevent/restore lung damage.
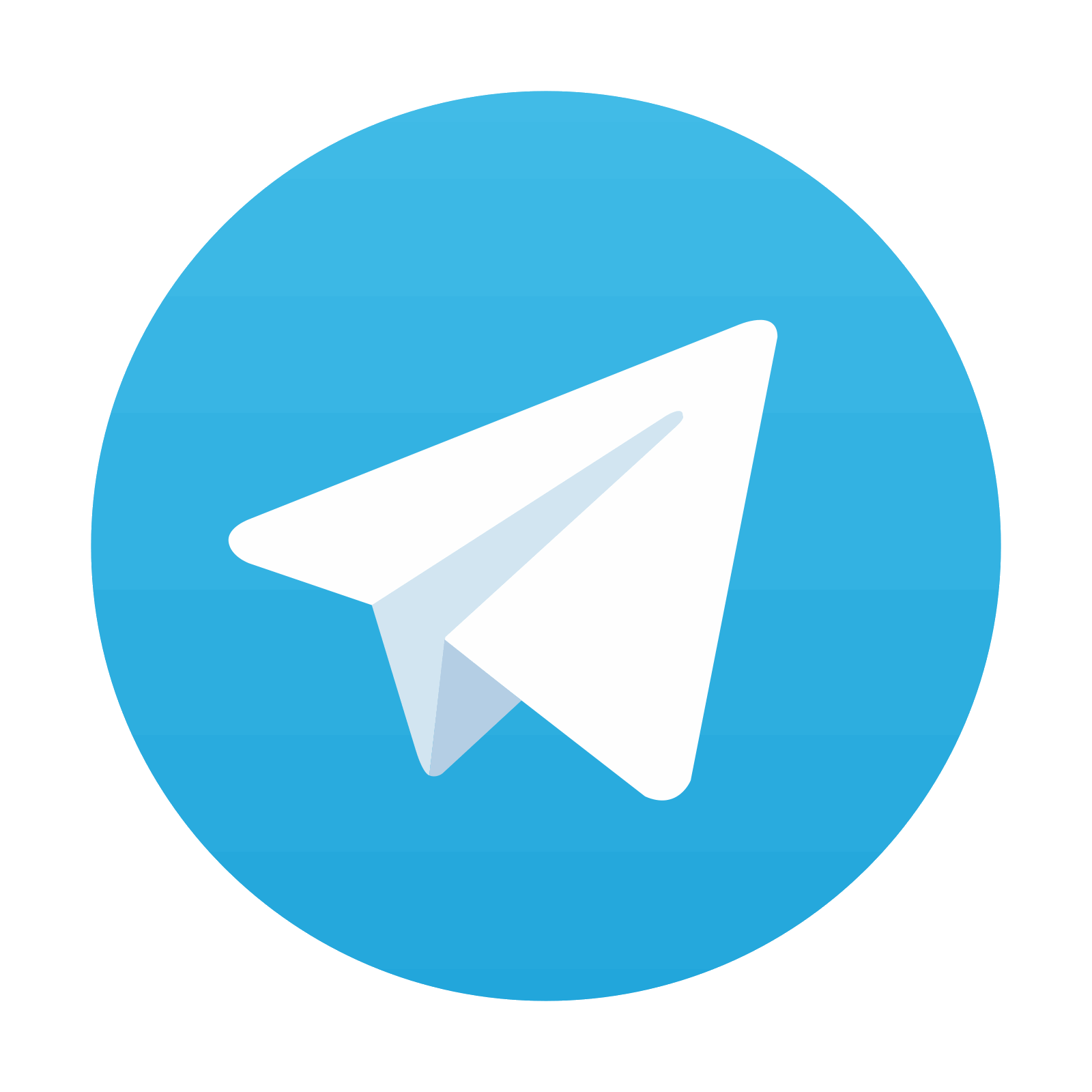
Stay updated, free articles. Join our Telegram channel
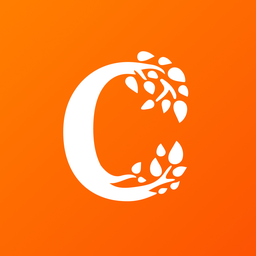
Full access? Get Clinical Tree
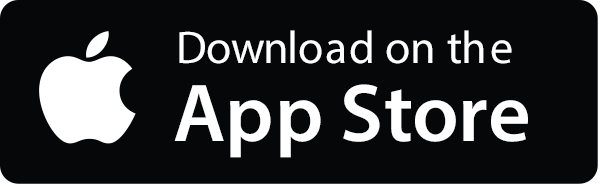
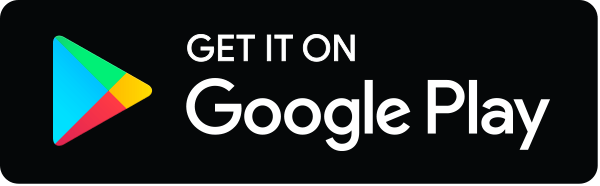