Chapter 15 Principles of Catheter Ablation
Ablative therapy for cardiac arrhythmias is based on the rationale that for every arrhythmia, a critical anatomic region of abnormal pulse generation or propagation is required for the arrhythmia to be initiated and sustained. Selective destruction of that area of myocardial tissue results in elimination of the arrhythmia. This principle was first demonstrated in 1968 in successful surgical division of a bundle of Kent in a patient with Wolff-Parkinson-White (WPW) syndrome.1 Since then, catheter-mediated ablative techniques have nearly completely replaced surgical procedures because of high clinical success rates and minimal morbidity associated with catheter techniques. Other chapters in this book deal with specific mapping and ablation techniques; this chapter deals specifically with the conceptual basis, biophysical principles, and pathologic lesions of catheter ablation.
Historical Background
The first catheter techniques of ablation used direct current with a conventional external defibrillator to deliver energy between the catheter placed adjacent to the cardiac structure targeted and an adhesive electrode with a large surface area applied to the skin. The first reported targeted use of catheter ablation for elimination of atrioventricular (AV) conduction in humans was in 1982, although accidental ablation had been reported by Vedel in 1979.2,3 Although direct-current shock was effective for ablation, significant complications related to barotrauma occurred.4 Catheter-based ablative techniques did not gain widespread acceptance until the advent of radiofrequency (RF) ablation. The first clinical application of RF current occurred when Harvey Cushing, working with W.T. Bovie, used high-frequency current for electrocoagulation.5 Since then, multiple animal studies have evaluated the influence of electrode size, electrode-tissue contact pressure, pulse power, duration, impedance, and temperature.6–8 In vivo observations were made in animal models with the use of RF energy in the ventricles, the atrium, the coronary sinus, and the tricuspid annulus.9–16 These observations were critical to the successful application in humans reported in the early 1990s.17,18
Radiofrequency Ablation
Biophysical Aspects of Radiofrequency Ablation
RF energy spans a wide range of frequencies, exhibiting a varying combination of resistive and dielectric properties. The RF band of 300 to 30,000 kHz is used for coagulation, ablation, and cauterization of tissues in medicine. Frequencies lower than 100 kHz stimulate excitable muscular tissue as well as cardiac tissue, resulting in muscle contractions and pain. Frequencies greater than 1000 kHz are effective in generating tissue heating; however, as frequencies increase, the losses along the transmission line increase, and the mode of heating changes from resistive heating to dielectric heating.19 Therefore, for ease of application, safety, and patient comfort, most standard RF generators produce signals in the range of 300 to 750 kHz.20
Application of RF current to the myocardium results in the generation of heat because of resistive losses in a di-electric medium (Figure 15-1). The magnitude of heating is proportional to the power density within tissue, which diminishes in proportion to the radius to the fourth power. Because of this property, only a small volume of myocardial tissue is heated directly by the RF energy (<2 mm in most cases), whereas the remaining tissue heating occurs via conduction.8 Haines and Watson have developed a simplified thermodynamic model to study the dynamics of heat transfer within a uniform medium at steady state. In this model, tissue temperature falls in an inverse proportion to the distance from the heat source (Figure 15-2). It also predicts that when the temperature at the electrode-tissue interface is maintained at a constant by varying the power, the lesion size should be proportional to the temperature and to the electrode radius.8 Clinical studies have demonstrated the association of higher electrode-tissue temperature and larger tip electrodes with larger RF lesion size and procedure efficacy.17,21 However, the temperature of the subendocardial tissue can exceed 100° C and result in rapid steam expansion and crater formation, often accompanied by an audible popping sound.7 These so-called pop lesions may be associated with coagulum formation on the RF ablation electrode and result in thrombogenicity, or these lesions may result in rupture, particularly of thin-walled structures such as atria.
In general, RF lesions are created by application in a unipolar fashion. Current passes between the tip of an ablating electrode in contact with the myocardium and a grounded reference patch electrode placed externally on the patient’s skin.22 Because RF current is an alternating current, the polarity of connections from the electrodes to the generator is unimportant. The surface area of the standard ablating electrode (approximately 12 mm2) is significantly smaller than the surface area of the dispersive electrode (100 to 250 cm2), and thus the power density and resultant tissue heating are highest at the electrode-tissue contact point, and no substantive heating occurs at the dispersive skin electrode.
The temperature rise at the electrode-tissue interface is rapid (t1/2, 7 to 10 seconds). However, thermal conduction to deeper tissue layers occurs more slowly, resulting in a steep tissue gradient. Studies have shown that steady-state lesion size is not achieved until after 40 seconds of ablation and that intramyocardial temperature rises steeply until it reaches a plateau at 60 seconds, with the majority of heating occurring within the first 30 seconds (Figure 15-3).23 However, because heating of the deeper layers is delayed, tissue temperature will continue to rise in the deeper layers despite the termination of energy delivery.24 This thermal latency phenomenon may be responsible for the progression of the undesirable effects of ablation such as AV nodal block despite prompt cessation of energy delivery in clinical situations.
Clinically, the efficacy of RF ablation depends on precise target site mapping and adequate lesion formation.21,25,26 A wide range of experimental and clinical observations support the notion that applied energy, power, and current are not precise indicators of the extent of lesion formation.27 By contrast, actual electrode-tissue interface temperature remains the best predictor of actual lesion volume.23 Typically, 55° C to 65° C is the target temperature for many temperature control catheters used clinically.28,29 If the temperature is lower than 50° C, none or minimal tissue necrosis is expected. However, excessive heating leads to coagulum formation and limitation of lesion size.
A number of clinically available RF ablation systems use temperature monitoring during ablation to prevent the formation of coagulum and achieve adequate electrode–myocardial tissue temperature. They either use a thermocouple or a thermistor placed in the catheter tip.30 A thermocouple is composed of two metals that are in contact with each other and generate a small current proportional to ambient temperature. Typically, thermocouples are placed within the catheter tip in contact with the distal electrode. A thermistor is essentially a semiconductor, which has an intrinsic resistance that changes in a predictable fashion with temperature. Small thermistors can be incorporated into a catheter tip and are thermally isolated from the shredding tissue with an insulating sleeve.30 Currently, such catheters are used as part of a temperature monitoring, closed-loop feedback system, in which the RF generator automatically adjusts the power to maintain a user program temperature. Clinical use of temperature monitoring has resulted in a decreased incidence of coagulum formation during ablation.25
Numerous limitations may result in discrepancies between the recorded temperature and the true electrode–myocardial tissue temperature. The catheter electrode may not be in intimate contact with the myocardium at the site of the thermistor or thermocouple. This may underestimate the myocardial interface temperature, particularly at sites where the catheter electrode may be parallel, rather than perpendicular, to the endocardial surface, such as occurs with accessory pathway ablation via the trans-septal approach.31 In addition, temperature monitoring becomes difficult as the electrode size increases or the electrode geometry changes. As the electrode size increases, the maximal temperature may be greatly underestimated by measuring just the tip temperature by a single-point thermocouple. Also, in longer electrodes used for linear ablation, the temperature at the edges may exceed the temperature in the electrode body, a phenomenon called the edge effect.32
Pathologic Aspects of Radiofrequency Ablation
Research using mammalian cell culture lines has shown that tissue survival during hyperthermia is a function of both temperature and time.33 Higher temperatures lead to a more rapid and greater degree of cell death. In vitro studies have shown that irreversible myocardial cell death during conventional ablation likely occurs between 52° C and 55° C.34 Typically, RF catheter ablation results in a high temperature (55° C to 60° C) for a relatively short time (30 to 60 seconds). In tissue immediately adjacent to the electrode, rapid tissue injury occurs, but in tissue heated by conductive heating, a relatively delayed myocardial injury occurs, with increasing distance from the RF electrode.7 Clinical outcome during catheter ablation has been shown to correlate with maximum tissue temperature achieved, with a temperature of 62° C ± 15° C associated with irreversible electrophysiological effects and a temperature of 50° C ± 15° C associated with reversible electrophysiological effects.21
The hyperthermia induced by RF energy has immediate and profound effects on cellular electrophysiology, structure, and function. These include the cellular effects on plasma membrane, the cytoskeleton, the nucleus, and cellular metabolism. Exposure to high temperatures results in inhibition of membrane transport proteins and structural changes to ion channels and ion pumps.35,37,38 Thermal effects on the cytoskeleton include disruption of stress elements, loss of plasma membrane support, and membrane blebbing.39,40 Nuclear structure and function are lost.41 With denaturation of metabolic proteins, cellular metabolism is inhibited.42 The microvasculature is damaged, and microvascular blood flow is reduced.43 At the level of the plasma membrane, transient nonspecific ion-permanent pores are formed in a plasma membrane.44 These effects cause an initial reversible loss of conduction immediately after the initiation of RF energy, which is likely caused by an electrotonic-induced and heat-induced cellular depolarization. Irreversible loss of electrophysiological function occurs immediately after successful ablation; this is likely caused by thermal tissue injury, which results in focal coagulation necrosis. A secondary inflammatory response and ischemia occur as a consequence of microvascular damage, which may cause progression of tissue injury within the border zone.43 Alternatively, in approximately 5% to 10% of cases, the RF-induced tissue injury resolves within the border zone, leading to late recovery of electrophysiology function and arrhythmia recurrence.
Determinants of Lesion Size
RF energy produces lesions that are homogeneous and well demarcated from surrounding tissue (Figure 15-4).
The lesion size in RF ablation is affected by several factors, including electrode configuration and size, power, duration, temperature, and myocardial tissue properties.45 The shape of the electrode can affect the size of the RF lesion by affecting the current density. Although needle-shaped electrodes have yielded the largest lesion size, dome-shaped electrodes are in use clinically because of their practical design.46 Electrode size has also been shown to be an important determinant of the lesion size in RF ablation. With increasing electrode size, the lesion size increases, as long as the electrode surface maintains contact with the endocardial surface and power is used to maintain the current density.47 Increasing the electrode size at some point may decrease the lesion size when a significant proportion of the electrode is not in contact with the myocardium, and thus energy is dissipated into the blood pool. Studies have shown that lesion size is nearly doubled with 4-mm electrodes compared with 2 mm electrodes. Similarly, lesion size continues to increase as the tip electrode size is increased from 4 mm to 8 mm but decreases with larger electrode sizes.48 In addition, significant char and coagulation formation is observed with electrode size greater than 8 mm.
With increasing duration of energy delivery, the lesion size increases but then reaches a plateau. In vitro studies have shown that in RF ablation, lesion size increases within the first 30 seconds, but the rate of increase significantly diminishes with extended power delivery.34 Similarly, lesion size increases as the temperature increases. However, as discussed earlier, when the temperature becomes excessive, the incidence of coagulation formation increases, thus impairing the ability to increase lesion size further. The coupling of RF energy to the endocardial surface is enhanced by improved contact of the electrode with the myocardium.23 Instability of the catheter electrode results in fluctuating temperatures because of convective loss of energy resulting in poor tissue heating. The efficiency of energy transfer between the RF catheter tip and the endocardium is also dependent on catheter-tissue orientation and the angle of the electrode contact. With perpendicular or oblique placement, lower temperatures are achieved compared with parallel electrode orientation.49,50
Cooled Radiofrequency Ablation
A technologic development that uses the fundamentals of RF-energy but improves its method of delivery is cooled RF ablation. Cooling of the ablation electrode tip has been shown to reduce both overheating of the electrode–tissue interface and impedance rise during RF delivery, which allows for greater power delivery. During cooled RF ablation, maximum temperature is achieved just below the endocardial surface, which translates into a deeper lesion.51–53 Since the catheter irrigant cools the tip-tissue interface, the measured catheter tip temperature will have an even greater discrepancy with tissue temperature compared with standard RF delivery.54 As a result, lower maximal electrode temperatures are imposed during RF delivery.55
The tip of the catheter can be cooled either by using a closed-loop (internal) design, in which saline is circulated through the catheter lumen and the ablation electrode, or by an open (external) design, in which saline is irrigated via small holes at the tip of the electrode (Figure 15-5). In the power-controlled mode, power settings range from 20 W to a maximum of 50 W with infusion rates more than 17 mL/min and 36 mL/min for external and internal irrigation, respectively. Saline flow rates and tissue contact pressure also affect the size of the lesion.56 A balance between high flow rates exists, resulting in a larger lesion and potential waste of RF current caused by overcooling. Contact pressure has also been shown to affect lesion diameter and thrombus formation. A deeper lesion is achieved with high catheter pressure, particularly in a perpendicular position, as less heat is lost to the blood pool and more energy is transmitted to the tissue. As a result, in locations where high contact pressure is readily achieved, such as the coronary sinus, power output levels should be reduced to less than 30 W to avoid complications.56 When a resistant site to ablation is encountered, increasing the duration of ablation, rather than applying higher power, may be a better choice for increasing lesion depth.57 Although open-loop systems do not completely prevent the risk of impedance rise and popping, Yokoyama et al demonstrated, in an animal model, that an open-loop system, compared with a closed-loop system, results in lower incidences of thrombus formation as well as steam pops.58,59
Cooled RF ablation has been shown to be effective and safe in patients with a variety of arrhythmias, particularly atrial flutter (AFL), atrial fibrillation (AF), and ventricular tachycardia (VT).60–62 In a canine model, Gerstenfeld et al performed pulmonary vein isolation and compared the histopathology of the lesions of an 8-mm tip standard RF catheter at 70 W, 20 seconds, 60° C and 50 W, 60 seconds, 50° C with a 3.5-mm irrigated tip ablation catheter at 35 W, 60 seconds, 45° C. The irrigated tip lesions resulted in less endocardial eschar formation, pulmonary vein (PV) stenosis, or damage to collateral structures.63 In clinical trials, an open irrigated tip catheter has been shown to be more efficacious than a conventional 4-mm tip catheter with respect to AF recurrence.64 Cooled RF ablation has also been extensively evaluated in single-center and multi-center studies of the ablation of VT.62 Soejima et al revealed a superior efficacy of cooled tip (89%) versus standard RF energy (54%) (P = .003) in the termination of VT at isthmus sites where an isolated potential was present.65 The largest trial, to date, of catheter ablation for recurrent monomorphic VT after myocardial infarction (MI) is the Thermocool VT trial.66 Ablation abolished all inducible VTs in 49% of patients. The procedure mortality rate was 3%, with no strokes.
The ability of cooled RF energy to treat VT from an epicardial approach has also been demonstrated. In a canine model, it was shown that standard techniques of RF ablation are not sufficient to create lesions in the pericardial space where convective cooling by blood is lacking. Fenelon et al demonstrated that an irrigated 4-mm tip catheter, compared with a standard RF 4-mm tip catheter, can deliver high power outputs, 43 W versus 16 W, and deeper lesions, 6.4 mm versus 3 mm, respectively.67 Whether endocardial RF or epicardial RF energy is delivered, a caveat to the application of external irrigation is that for the administration of a potentially significant volume of fluid, peri-procedural precautions should be taken to manage the volume load.
Cryoablation
Cryoablation is unique in that it causes tissue injury by freezing and thawing rather than by hyperthermia. This mode of ablation results in minimal tissue destruction and preserves the basic underlying tissue architecture. A large body of clinical experience has been accumulated in the use of cryothermy in the surgical treatment of tachyarrhythmias, in which it has been proven to be safe and effective. Cryoablation has been used extensively intraoperatively for the creation of AV block or ablation of atrial tachycardia (AT) and VT.68,69 In 1998, the first percutaneous catheter ablation using cryothermal energy was performed.70
One advantage of cryothermal energy is that it also allows for reversible “ice mapping,” in which the area likely responsible for the arrhythmia can be evaluated by suppressing its electrophysiological properties before the creation of an irreversible state.71 During cryomapping, reversible cooling is performed at tissue temperatures of approximately −28° C to −32° C without tissue destruction, whereas irreversible lesions are accomplished by achieving temperatures of less than −60° C for 2 or more minutes. The current guideline to limit cryoablation time to 4 minutes is based on the fact that the ice ball has been shown, via ultrasound visualization, to enlarge during the first 3 minutes of the freezing cycle and then remain stable.72,73
Application of a cryoprobe to a tissue surface results in the formation of a well-demarcated hemispherical block of frozen tissue. At the cellular level, intracellular and extracellular ice crystals form, followed by thawing, which further disrupts membranous organelles and enhances the lesion. Within 24 hours, coagulation necrosis results, with hemorrhage, edema, and inflammation. Over time, the lesion becomes sharply demarcated from surrounding tissue and is finally replaced by fatty and fibrous tissue in 2 to 4 weeks (Figure 15-6).74 The lesion of cryoenergy is significantly less thrombogenic than that of RF energy, as a result of the preservation of endothelial cells and tissue ultrastructure.75
Several different catheter-based designs have been developed for the cryoablation of arrhythmias (Figure 15-7).76 They differ among the techniques employed to achieve freezing temperatures. Some designs are based on the Joule Thompson effect, in which a pressure drop results in significant cooling or freezing, or on the Peltier effect (thermoelectric effect), in which a temperature difference is created by applying a voltage between two electrodes. Gases such as nitrous oxide or halocarbon are used as refrigerants. The size of the cryoablative lesion depends on various factors such as temperature, duration, surface area of the cooling probe, and the number of applications.
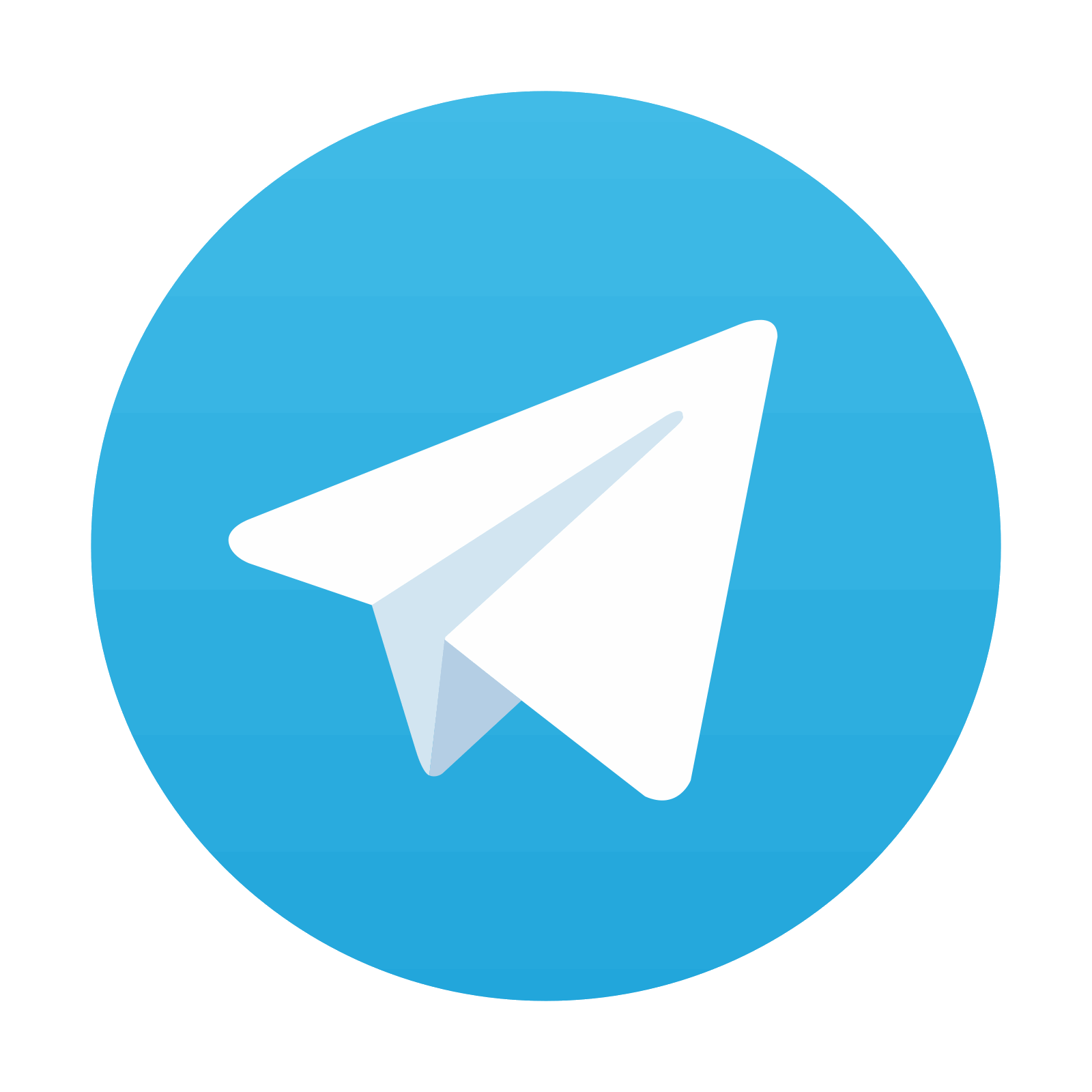
Stay updated, free articles. Join our Telegram channel
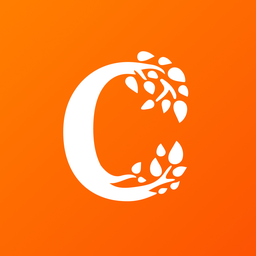
Full access? Get Clinical Tree
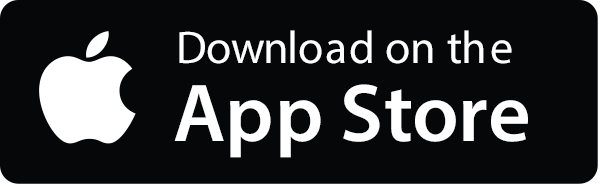
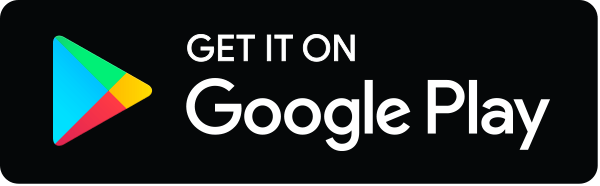