It would be only partially correct to state that the respiratory system is the human physiologic system responsible for cellular oxygenation. Likewise, it would be false to state that the cardiovascular system assumes full responsibility for cellular oxygenation in the body. Neither of these systems alone can accomplish this life-sustaining function. Rather, it is the combined, cooperative effort of these two systems that is required. Thus, it is valid, both conceptually and clinically, to view these two systems as a single, integrated cardiopulmonary system that works to accomplish the ultimate goal of tissue oxygenation and carbon dioxide (CO2) excretion (Fig. 6-1). Traditionally, the complete physiologic process of cellular oxygenation and the work of the cardiopulmonary system have been divided into three steps or phases (Fig. 6-2). In step one, ambient O2 molecules are moved from their atmospheric origin to the blood supply within the lungs. O2 actually enters the circulatory system via the small blood vessels in the lungs (pulmonary capillaries). O2 molecules diffuse into the blood from the tiny air sacs in the lung known as alveoli. The exchange of O2 and CO2 between the alveoli and pulmonary capillaries is called external respiration and is the essence of step one (see Fig. 6-2,A). Step two involves the quantitative transport of a sufficient volume of O2 from the pulmonary capillaries to its cellular destination. This process, which is commonly referred to as O2 transport, requires a normal hemoglobin concentration as well as an adequate cardiac output. Cardiac output may be defined as the volume of blood ejected each minute from the heart. The assessment of the adequacy of step two is generally quantitative (i.e., Is a sufficient volume of O2 being delivered to the tissues?). This step is depicted in Figure 6-2,B. The final link in the O2 delivery chain is the diffusion of O2 from small systemic capillaries in response to cellular metabolic needs. This step, called internal respiration, involves both the diffusion of O2 to the cells and its metabolic utilization by the cells (see Fig. 6-2,C). Internal respiration is defined technically as the exchange of O2 and CO2 between the systemic capillaries and the cells or tissues. In this text, however, the actual metabolism that occurs in the cells is also considered to be part of the process of internal respiration. The common link throughout this O2delivery system is the blood and specifically the hemoglobin within the blood. The blood plays a pivotal role in all three phases. By using the blood or hemoglobin as a focal point, the three steps in the delivery of O2 can be thought of as simply: O2 loading (see Fig. 6-2,A), O2 transport (see Fig. 6-2,B), and O2 unloading (see Fig. 6-2,C). The remainder of this chapter will address the first step in oxygenation, external respiration or oxygen loading (see Fig. 6-2,A). The normal function and possible pathologic changes that can occur to disrupt external respiration will be explored. Three criteria must be met to ensure adequate O2 loading via external respiration (Fig. 6-3). First, an ample supply of O2 must reach the alveoli, which depends mainly on the adequacy of ventilation. Ventilation is the gross movement of air into and out of the lungs. The volume of blood flow is not uniform throughout all lung segments. Rather, perfusion is preferentially distributed to gravity-dependent lung regions. Thus, in a man or woman placed in an upright position, the lung bases receive the largest proportion of the cardiac output, whereas the lung apices receive the least proportion. When lying supine (on the back), most blood goes to the posterior lung surface while the anterior (front) surface is minimally perfused (Fig. 6-4). West has described a three-zone conceptual model of pulmonary perfusion in which the general regulation and characteristics of perfusion are different in each zone155 (Fig. 6-5). Zone 1, when it is present, is always in the least gravity-dependent (uppermost) portion of the lung. Conversely, zone 3 is always in the most gravity-dependent (lowest) area of the lung. Of course, zone 2 is between the other two zones. Perfusion is absent in zone 1, sporadic in zone 2, and constant in zone 3. Zone 1 is a theoretical area of the lung where perfusion is nonexistent because pulmonary arterial pressure is less than alveolar pressure (PA>Pa); consequently, the pulmonary capillary is collapsed (Fig. 6-6). The pulmonary circulation is a low-pressure system (normal pulmonary artery pressure 25/10 mm Hg) and, therefore, there is not a great deal of force available to pump blood to the uppermost areas of the lungs. Zone 2 is a functional area where the flow of perfusion is moderate. In zone 2, pulmonary arterial pressure is greater than alveolar pressure and, therefore, flow through the capillary is initiated. Zone 2 is also characterized by an alveolar pressure that exceeds pulmonary venous pressure (Pa>PA>Pv). Thus, flow occurs in this area because pulmonary arterial pressure exceeds alveolar pressure. Furthermore, the amount of flow depends on the difference between the pulmonary arterial pressure and the alveolar pressure. Because the pulmonary arterial pressure is progressively higher as one moves toward the lower regions of the lung, there is likewise a progressive increase in perfusion as one moves down this zone (see Fig. 6-6). Zone 3 is the most gravity-dependent lung region in which blood flow is heavy and relatively constant. Zone 3 is characterized by a pulmonary venous pressure that exceeds alveolar pressure (Pa > Pv > PA).155 In zone 3, perfusion is based simply on the difference between arterial and venous pressure, and alveolar pressure is not important. The majority of pulmonary perfusion occurs in zone 3. Although the zone model may help one to understand the functional characteristics of pulmonary perfusion, it may sometimes be misleading. In the actual lung, there is no clear demarcation of lung perfusion zones. Rather, there is a general, linear increase in perfusion as one moves from the apex to the base of the lung (Fig. 6-7). The volume of gas remaining in the lungs following a normal exhalation is called the functional residual capacity (FRC) and is shown in Figure 6-8. At normal FRC, more gas resides in the upper lung zones (apices) and less in the lung bases. As shown in Figure 6-9, alveoli are larger in the apices and smaller in the bases. This regional variation in FRC volume can be explained by regional differences in transpulmonary pressure. Transpulmonary pressure (PL) is the difference in pressure across the lung. It is defined as the pressure inside the lung minus the pressure immediately outside the lung in the pleural space. At the alveolar level, transpulmonary pressure is equal to the pressure within the alveolus (PAlv) minus the intrapleural pressure (Ppl). Intrapleural pressure is the pressure within the pleural cavity that surrounds the lungs. Thus, the formula for calculating transpulmonary pressure is shown in Equation 6-1. It is common in the literature to refer to the normal intrapleural pressure at rest as a single negative number such as (-4 cm H2O).81 This is slightly misleading, however, because this single number is the average intrapleural pressure throughout the intrapleural space. Actually, the intrapleural pressure at the base of the lung is almost 8 cm H2O higher than that in the apex (Fig. 6-10).155 This increase is probably related to the increased blood present in the bases. Intrapleural pressure increases linearly at a rate of approximately 0.25 cm H2O for every centimeter of distance down the lung.155 Figure 6-10 shows the transpulmonary pressure across the alveoli in the lung apex compared with the transpulmonary pressure across the alveoli in the lung base at normal resting lung volume. As additional air is added to the lung beyond FRC (i.e., tidal volume [VT]), it will preferentially ventilate the lung bases. At normal FRC, compliance of basilar alveoli is greater than compliance of apical alveoli, which are more distended. Thus, most of the gas inhaled during normal breathing actually ventilates the bases (see Fig. 6-9,B). In addition, the lower intercostal muscles and the diaphragm are displaced more than the upper part of the chest during normal inspiration, which may further facilitate basilar expansion.156 The actual distribution of tidal ventilation in the upright lung is shown in Figure 6-11. Clearly, ventilation is greatest in the lung bases. On the other hand, if one inhales more deeply than usual (large VT), and particularly when inspiratory hold is used, VT is distributed more evenly throughout the entire lungs.156 The normal distribution of perfusion is shown in Figure 6-12,A. A number of factors are known to alter this normal pattern of pulmonary perfusion. For convenience, these mechanisms are classified as primary or compensatory mechanisms. Primary disturbances are simply pathologic changes in pulmonary perfusion. Compensatory disturbances are changes in the pattern of pulmonary perfusion in response to a change in pulmonary ventilation. Compensatory changes attempt to improve or to restore ventilation-perfusion matching. Primary disturbances of perfusion may be localized or generalized. Serious local primary disturbances may be caused by pulmonary emboli or vascular tumors that affect the pattern of perfusion. Drugs such as isoproterenol, nitroglycerin, or propranolol may also alter the pattern of perfusion and may affect the PaO2.157 158 Most commonly, however, primary disturbances are the result of a generalized increase or decrease in pulmonary perfusion. A generalized increase in pulmonary perfusion tends to move the borders of the perfusion zones upward and has an overall tendency to distribute perfusion more equally throughout the entire lung (see Fig. 6-12,B). The volume of blood present in the lungs may be increased because a greater amount is pumped to the lungs from the right side of the heart (e.g., increased cardiac output). Alternatively, pulmonary blood volume may be increased due to backpressure from poor left-sided heart function (e.g., mitral stenosis, left-sided heart failure) and pooling of blood in the lungs. Conversely, a generalized decrease in pulmonary perfusion results if the cardiac output decreases due to inadequate blood volume or heart (pump) failure. A decrease in the quantity of pulmonary perfusion causes the upper margins of the lung zones to move downward (see Fig. 6-12,C), which, in turn, may precipitate the development of a zone 1 area where ventilation is present without perfusion. It is noteworthy that the application of positive pressure ventilation may be associated with a similar shifting of the pulmonary perfusion zones downward. Overall, pulmonary perfusion could likewise decrease if the pulmonary blood vessels constrict (increased pulmonary vascular resistance) and the heart is unable to pump blood throughout the entire lung (see Fig. 6-12,D). Normally, increased pulmonary vascular resistance (PVR) is countered with an increased right-sided heart pumping force. Thus, the normal distribution of pulmonary perfusion is usually maintained despite an increase in PVR. However, when the heart is unable to increase its pumping force because it is weak or damaged, increased PVR may result in a generalized decrease in perfusion. PVR may increase acutely due to hypoxemia or acidemia. Remarkably, the pulmonary vessels are the only blood vessels in the body that react to low O2 levels by constriction rather than dilation, although the reason for this is still unclear.159 160 It can also be shown on a macroscopic level that as lung volume decreases, relatively more perfusion is distributed to nondependent lung regions. If FRC is allowed to fall completely to residual volume (RV), blood flow is actually greater at the second rib level than at the lung bases in an upright person.155 Again, this appears to maximize the ventilation-perfusion interface because at low lung volume the distribution of ventilation is similar. Furthermore, because dependent lung zones are particularly prone to pathologic alveolar collapse or consolidation, an upward shift of perfusion in these situations seems to be especially beneficial. On a local level, the partial pressure of O2 in the alveoli (PAO2) serves as the primary regulatory mechanism.158 Decreases in PAO2 that result from poor ventilation to a specific lung area result in profound arteriolar and venule constriction and thus minimize perfusion to a poorly ventilated space (Fig. 6-13,A). The release of histamine from hypoxic mast cells has been suggested as a potential mediator of this response,156 but regardless of the mechanism, the net effect is to improve the ventilation-perfusion match. The single most common cause of abnormal distribution of ventilation is increased pulmonary secretions. The accumulation of secretions leads to decreased airway diameter and turbulent gas flow, both of which increase airway resistance. Other causes of increased airway resistance include bronchospasm, mucosal edema, artificial airways, and external compression of the airways by an abnormal tumor or fluid space. The effects of increased secretions or bronchospasm in the lower airway on the distribution of ventilation are shown in Figure 6-14,A. An abnormal FRC also leads to the abnormal distribution of ventilation, which is true regardless of whether the FRC is increased or decreased. Both situations lead to changes in alveolar compliances throughout the lung and changes in the distribution of inspired gas. The effect of atelectasis and a decreased FRC on the distribution of ventilation is shown in Figure 6-14,B. The application of positive-pressure ventilation disturbs the normal distribution of ventilation (see Fig. 6-14,C). Positive-pressure ventilation increases the distribution of ventilation to upper lung zones while simultaneously decreasing perfusion to these areas. Thus, the application of mechanical ventilation interferes with ventilation-perfusion matching and normal external respiration. Finally, a less recognized clinical problem in ventilation distribution is the phenomenon of airway closure. When the lung is compressed, such as during forced expiration, a point in the expiratory phase can be shown at which gravity-dependent lung zones cease to ventilate (see Figure 6-14,D). Dependent lung regions are the lung zones that are most affected by gravity. The actual anatomic location of these regions varies with body position. Regional airway collapse during forced expiration was the basis for the closing volume study, a pulmonary diagnostic test that gained popularity in the 1970s for its purported ability to detect lung disease at a very early stage.161 It was speculated that individual knowledge of the presence of early lung disease (i.e., premature airway closure) would serve as a deterrent to smoking. However, no data are available to substantiate this claim. In healthy young individuals, airway closure does not occur until very near residual volume (RV) and in some is not seen at all. RV is, of course, the volume of gas remaining in the lungs after maximal expiration. In certain individuals (e.g., the elderly, children, obese, and smokers) and particularly in the presence of certain predisposing factors (e.g., reduced bronchial muscle tone, small airway disease, pulmonary edema, decreased elastic recoil in lungs, forced expiration), airway closure occurs at much higher lung volume.161 162 163 In fact, basal airway closure above FRC is common in patients with pulmonary emphysema.155 Of clinical concern, airway closure may occur in susceptible individuals during normal tidal ventilation, particularly when the FRC is reduced. The FRC, in turn, has been reported as decreased in the following: supine position, under anesthesia,164 pain, obesity, smoking, and prolonged bedrest.165 Simple assumption of the supine position may in itself decrease FRC (300 to 800 mL).165 Thus, in individuals prone to airway closure or in those with diminished FRC, the clinician should strongly suspect this gas exchange problem. In healthy individuals older than 65 years of age, airway closure during tidal ventilation is likely to occur.165 Furthermore, the decrease in FRC associated with the supine position would allow this to happen at 44 years of age in healthy people.165
Oxygenation and External Respiration
INTRODUCTION
Cardiopulmonary System
Steps in Tissue Oxygenation
EXTERNAL RESPIRATION
Ventilation-Perfusion Matching
Normal Distribution of Pulmonary Perfusion
Gravity Dependence
West’s Zone Model
Zone 1.
Zone 2.
Zone 3.
Normal Distribution of Ventilation
Gas Distribution at Functional Residual Capacity
Equation 6-1
Normal Distribution of Tidal Volume
Abnormal Distribution of Pulmonary Perfusion
Primary Disturbances
Generalized Increase in Pulmonary Perfusion
Generalized Decrease in Pulmonary Perfusion
Macroscopic Changes
Local Changes
Abnormal Distribution of Ventilation
Primary Disturbances
Increased Airway Resistance.
Abnormal Functional Residual Capacity.
Positive-Pressure Ventilation.
Airway Closure.
Stay updated, free articles. Join our Telegram channel
Full access? Get Clinical Tree
Oxygenation and External Respiration
Only gold members can continue reading. Log In or Register a > to continue
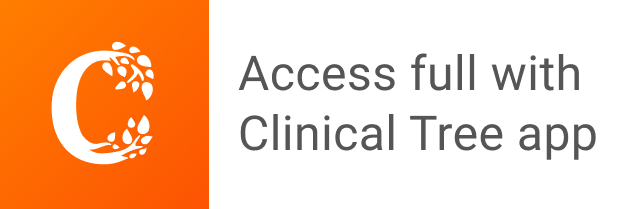