56 Optogenetics is an emerging technology for optical interrogation and control of biological function with high specificity and spatiotemporal resolution. Mammalian cells and tissues can be sensitized to respond to light by a simple and well-tolerated genetic modification using microbial opsins (light-gated ion channels and pumps). Fast and specific excitatory or inhibitory responses can be achieved, with distinct advantages over traditional pharmacologic or electrical perturbation. The breakthrough came with the discovery of fast microbial opsins that behave like gated ion channels,1,2 and the subsequent demonstration that these microbial opsins (channelrhodopsin2, ChR2, in particular) can generate sufficient photocurrent to optically stimulate and control mammalian neurons with very high temporal resolution.3,4 Optogenetics has already been widely used to elucidate brain circuitry and function in health and disease,5–7 yet expansion of this emerging technology in cardiovascular research has surprisingly remained largely unexplored. Bacteriorhodopsin (BR) is one of the simplest and best studied optoelectrical transducers from the microbial (class I) opsins, found in archae, eubacteria, fungi, and algae. It is a protein with seven transmembrane domains that acts like a light-gated active ion pump—it captures photon energy via its covalently bound chromophore, retinal—and moves protons against their electrochemical gradient from the cytoplasm to the extracellular space. Since their discovery,8 the microbial opsins have been viewed as potential components for bioelectronics and a new generation of optical memory because of their ultra-fine spatiotemporal control by light (i.e., their ability to address single molecules by focused light at very fast rates). The latter is of equal interest in control of eukaryotic cells. Structurally and functionally, BR provides good insight for optogenetics as it shares high homology with all (class I) opsins currently in use. Current-day optogenetics began with the characterization and cloning of ChR1 and the higher-conductance light-sensitive ion channel ChR2 from green algae by Nagel, Hegemann, Bamberg, and colleagues1,2 in 2002 and 2003. This was followed in 2005 by the first robust demonstrations of the use of ChR2 to stimulate mammalian cells.3,4 Upon heterologous expression, these microbial ion channels provide excitatory (cation-mediated) current with relatively fast kinetics9 and can effectively trigger electrical impulses (action potentials) in excitable cells upon light stimulation at relevant physiological rates. This demonstrated usefulness in neuroscience revived interest in other types of microbial opsins, discovered earlier and extensively studied within the microbial photobiology field. These include the chloride pump halorhodopsin (HR)10 and the BR-like proton pump archaerhodopsin (AR).11 Both have proved capable of providing outward/hyperpolarizing current in mammalian cells.12,13 What makes recent optogenetic tools (several types of microbial opsins) more practical compared with earlier systems and compared with classic (electrical and chemical) ways of stimulation are the following distinguishing characteristics: (1) simplicity of expression and operation without exogenous cofactors, offering the attraction of a single-component system, in contrast to prior multi-component optical actuators, such as using G-protein–coupled signaling and ligand-requiring opsins (chARGe)14; (2) apparent minimal interference with endogenous function15 (much less than genetically encoded voltage and calcium sensors) and remarkable reliability of use on a large scale in vitro and in vivo; (3) high specificity compared with electrical stimulation (i.e., selective cell type targeting due to the genetic means of manipulation); (4) very high spatiotemporal precision of manipulation (i.e., fast addressing of single molecules or cells by the combination of focused light and genetically defined cell targets; (5) robustness and range of action within the same paradigm (i.e., excitatory and inhibitory effects can be encoded); (6) lower light energy required for activation compared with prior attempts to stimulate by infrared light alone16; and (7) noncontact stimulation with precise localization, allowing highly parallel/high-throughput operations. Neuroscience applications have successfully used the specificity offered by optogenetics to dissect neural circuits and connectivity, linking specific neuron populations to normal behaviors and to disease. These studies include applications to better understand learning,17 olfactory processing in vivo,18 depression,19 sleep disorders,20 fear,21 and addiction.22 More translational studies tackled questions related to epilepsy and terminating seizures using inhibitory opsins,23 controlling Parkinson’s disease with deep brain stimulation (DBS),24,25 countering visual degeneration in retinitis pigmentosa,26 restoring respiratory control,27 optimizing nerve stimulation of skeletal muscle,28 and optimizing stem cell differentiation while taking advantage of the inherently parallel nature of optical stimulation.29 This is a small subset of the wide spectrum of studies conducted thus far; more recent and comprehensive reviews provide further information.6,7 ChR2 from Chlamydomonas reinhardtii, cloned by Nagel et al. in 2003,2 is the prototypical and most widely used optogenetic tool. Like BR, it belongs to class I microbial opsins, all of which require the cytosolic presence of retinal, which acts as a chromophore (light-sensing element). Unlike BR, ChR2 is an ion channel (not a pump), and upon opening it conducts cations down their electrochemical gradient. The chromophore, all-trans-retinal, is covalently bound to the ion channel, and the complex does not undergo the dissociation seen for class II mammalian rhodopsins, where the retinal/opsin complex is reassembled/disassembled upon each stimulus. Upon interaction with a photon, all-trans-retinal undergoes isomerization to 13-cis-retinal, triggering channel opening. All-trans-retinal, derived from intake of vitamin A–containing nutrients, is present only in small amounts in nonretinal and nonembryonic tissues (<0.5 nmol/g).30 It was a serendipitous finding that in most vertebrate cells, enough all-trans-retinal is present naturally to form functional ChR2 complexes. This is even more surprising in cell culture, where the source of vitamin A must be serum/cell culture impurities. To date, no systematic studies have demonstrated if and how retinal availability varies between cell and tissue types, and whether it can be a limiting factor in the light responsiveness of different cell types modified with ChR2. Similar to BR, ChR2 has seven transmembrane (TM) domains. It has a molecular weight of 77 kDa and a total of 737 amino acids, approximately 300 of which are located at the amino-terminus and fully define its photocurrent generation.31 The crystal structure of ChR2 was recently solved,31 and this revealed that the conductive pore is defined by TM1, -2, -3, and -7, and that TM7 is critical for the interaction with retinal, while TM2 determines channel selectivity and conductance. ChR2 has a higher energy barrier for excitation than BR (energy is inversely proportional to the wavelength)—its spectral response peaks at around 470 nm (570 nm for BR). ChR2 conducts cations with differential selectivity in the following order (H+ > Na+ > K+ > Ca2+, …9; Figure 56-1). More specifically, PH/PNa = 1.062 × 106; PK/PNa = 0.427; PCa/PNa = 0.117. Thus, for physiological concentrations and membrane potentials, ChR2 provides predominantly Na+-mediated inward current. It has a reversal potential close to 0 mV, showing inward rectification (i.e., minimal outward current3,9,32–35). Several competing theories have been put forward for the mechanism of rectification, including that it is a single-channel property defined by an asymmetrical barrier33 or a macroscopic property resulting from the kinetics of multiple ion species interacting with the channel.35 Figure 56-1 Biophysical Properties of ChR2 Upon a light pulse with the proper wavelength (470 nm) and sufficient irradiance (in mW/mm2) for excitation, ChR2 generates current that peaks rapidly and then relaxes to a smaller steady state level (see Figure 56-1). Higher irradiance and more negative voltages speed the kinetics of both activation and relaxation. Even at room temperature, all time constants are <20 ms.32,34 The single-channel conductance for the wild-type ChR2 is relatively small, and the few reported values vary widely from 40 to 90 fS33,36 to 0.25 to 2.42 pS,9 depending on the method of estimation. For comparison, the Na+ channel conductance in muscle cells is as large as 18 pS.37 Zimmermann et al. used freeze-fracture electron microscopy (EM) and particle counting as well as whole cell conductance and capacitance measurements to estimate ChR2 density of expression (typical case) and found about 2000 channels/µm.2,36 If all the channels were opened simultaneously, the generated whole cell current would be between 400 pA/pF and 25 nA/pF. As is discussed later, one of the first ChR2 single-amino-acid mutants (H134R) was designed to increase conductance by two- to threefold compared with wild type, with minimal slowing of kinetics.3 Conceptual and quantitative understanding of the function of ChR2 is aided by recent mathematical models, as proposed by Hegemann and colleagues38 and modified by others.39 A four-state model is currently favored, with two open states (a high-conductance state and a low-conductance, light-adapted one) and two closed states. Photon absorption and isomerization of retinal constitutes a near-instantaneous process, so that ChR2 conformational changes, after light sensing, determine its photocurrent kinetics. Current models capture light dependence well but oversimplify voltage dependence. Opsins that produce inhibitory/hyperpolarizing current include the chloride pump HR from Natronomonas pharaonis adopted for mammalian use, eNpHR,12 and some BR-like proton pumps (e.g., Archaerhodopsin-3 [AR] from Halorubrum sodomense13) and the lower-wavelength-activated pump from the fungus Leptosphaeria maculans (Mac).13 AR is the most potent inhibitory opsin to date; compared with HR and Mac, it offers larger photocurrent and faster recovery from inactivation. Similar to HR and Mac, AR provides an outward current with an extremely negative reversal potential and only about a 20% drop in current when going from 0 to −120 mV. By its action, despite being an efficient proton pump, AR changes the H+ concentration (pH) minimally (i.e., the intracellular pH can increase by about 0.15 after 1 minute of continuous illumination).13 The turnover rate of AR is unknown but by whole cell current (1 nA induced by strong illumination in neurons13) is comparable in amplitude with the ChR2 current, most likely because of high density of expression, as is the case for most active pumps usually with an order of magnitude of higher density than ion channels.40 Having both excitatory and inhibitory optogenetics tools of comparable performance opens the possibility for optical control of membrane potential (i.e., “shaping” the action potentials and/or the frequency response of the system). A method for optimized tandem expression of excitatory and inhibitory opsins has been recently developed.41 Genetic engineering is currently used to expand and optimize available opsins in three important aspects: light sensitivity, speed, and spectral response. These efforts, carried out mainly in the laboratories of Deisseroth, Bamberg, Hegemann, Tsien, Boyden, and others, have resulted in ChR2 mutants with single-amino-acid substitutions, including the higher-conductance H134R,3 T159C,42 and ET/TC42; the Ca2+ permeable CatCh43 and the speed-optimized ChETA44; hybrids of ChR1 and ChR2 (ChIEF)9; and hybrids of ChR1 and VChR1 (C1V1) for red-shifted variants,45 among others. In most cases, optimization in one aspect (e.g., conductance) comes with a tradeoff in another aspect (e.g., speed). Extensive quantitative comparisons of genetically engineered opsins can be found in several excellent reviews.5,6,42 Other efforts to improve the optogenetics toolbox include reduction of toxicity seen with early use of these opsins, better membrane targeting, better cell specificity, and optimization of expression.46 Since 2010, publications have begun to appear that extend optogenetics to cardiac muscle. Arrenberg et al.47 used a zebra fish model to express both excitatory (ChR2) and inhibitory (HR) opsins. Using structured illumination, they demonstrated the use of optogenetics to spatially map the pacemaking region in zebra fish during development. They also presented a range of rhythm disorders that were triggered optically. Bruegmann et al.48 published the first cardiac mammalian application. They combined viral expression of a ChR2 variant with a CAG promoter in mouse embryonic stem cells (mESCs), with targeted differentiation and purification of ESC-derived cardiomyocytes for in vitro demonstration of optical pacing. Furthermore, they generated transgenic mice with cardiac ChR2 expression, in which normal rhythm was perturbed in vivo by light pulses, and focal arrhythmias were induced by long pulses. Simultaneously and independently, our group demonstrated a nonviral optogenetic approach.34
Optogenetic Control of Heart Muscle
Defining Optogenetics
Nature-Derived Optical Actuators
Bacteriorhodopsin
The New Generation of Single-Unit Optical Actuators
Distinct Advantages of Optogenetics
Brief Overview of Neuroscience Applications
The Optogenetics Toolbox
Excitatory/Depolarizing Opsins—Channelrhodopsin2
A, ChR2 is a light- and voltage-dependent ion channel; all-trans-retinal, intracellularly available and covalently bound to ChR2, acts like a chromophore (sensing photons) to facilitate ChR2 opening and the transport of cations with differential preference from H+ to Ca2+. B, The resultant current is predominantly inward/excitatory with a fast peak and a sustained component; ChR2 exhibits strong inward rectification with a reversal potential around 0 mV; shown are also selected traces for ChR2 current under different voltage clamps (inset) and irradiance levels (bottom); scale bars are 10 pA/pF and 100 ms. (Data for the sustained component shown from Jia Z, Valiunas V, Lu Z, et al: Stimulating cardiac muscle by light: Cardiac optogenetics by cell delivery. Circ Arrhythm Electrophysiol 4:753-760, 2011.)
Inhibitory/Hyperpolarizing Opsins
Optimization of Optical Actuators
Optical Control of Cardiac Function
Overview of Early Work in Cardiac Optogenetics
Stay updated, free articles. Join our Telegram channel
Full access? Get Clinical Tree
Optogenetic Control of Heart Muscle
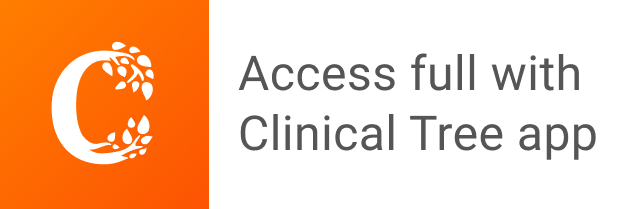