The technique of measuring the oxygen saturation of blood hemoglobin was described in 1932.590 Use of the term oximeter to describe the particular measurement device, however, was not introduced until 10 years later in 1942.591 Millikan (1906 to 1947) coined the term oximeter for the device that he invented to measure ear oxygen saturation.591 At that time, Millikan was working on the problem of aviators losing consciousness while at high altitude during battle. He solved this problem by inventing a servo-controlled oxygen supply system attached to an ear oximeter. Earlier, however, in 1860, invention of the spectroscope by Bunsen and Kirchhoff actually paved the way for the development of oximetry.591 The spectroscope was a device that was used initially to measure the exact wavelengths of light emitted after introducing elements into the flame generated by a Bunsen burner. Interestingly, each substance studied with the spectroscope had its own unique light emission spectrum. Apparently, each substance absorbed and therefore emitted light of different wavelengths in its own unique manner much like each individual has his or her own distinct fingerprints. The particular pattern of light absorption/emission at sequential light wavelengths can be graphed, and this pattern is known as the absorption spectrum of that particular substance. The absorption spectra of some of the more common forms of hemoglobin that may be present in the body are shown in Figure 15-1. Measurement of the light spectrum of an unknown substance may thus serve as a useful technique for qualitative analysis. Early techniques for actually measuring light intensity over sequential light wavelengths were difficult. For this reason, colorimetry, a simplified measurement technique that did not actually require measurement of light intensity, was often used in qualitative analysis. Colorimetry was a methodology wherein the color of a known substance was compared with that of an unknown substance.591 As such, colorimetry depended on visual acuity and perception and, consequently, was not highly exact. The current measurement technique of spectrophotometry is sometimes referred to incorrectly as a colorimetric method. This term is technically incorrect because color per se is not actually evaluated. Discovery of the photoelectric effect and development of practical photoelectric cells (photo detectors) paved the way for spectrophotometry as it is used today. The photoelectric effect is the ability of light to release electrons from metals in proportion to the intensity of the light (Figure 15-2). A photodetector can use this principle to measure light intensity and to convert it into electrical energy. In spectrophotometry, light is passed through a filter and is thus converted into a specific wavelength. This light is then passed through a cuvette that contains the substance being analyzed. The amount of light that passes through the cell is detected on the opposite side of the cuvette by a photo detector and is reflected on a meter (Figure 15-3). Thus, measurement of light emission at different wavelengths can be readily accomplished by using the technology of spectrophotometry. The term spectrophotometry (spectro-photo-metry) is based on the measurement (-metry) of light (-photo-) spectrums (spectro-). Quantitative spectrophotometry is made possible by application of the Lambert-Beer law. Io = intensity of light incident on the specimen Ix = intensity of the transmitted light k = a constant (characteristic of the substance and wavelength of the incident light) c = concentration of the absorbing substance d = pathlength in the absorbing medium (usually expressed in centimeters) During analysis of a substance, the ratio of light intensity incident on the substance (Io) is compared with the light intensity of the transmitted light (Ix). The ratio of Io/Ix is sometimes referred to as the optical density. Plotting out the optical density at various wavelengths leads to a graphic representation of the light absorption spectrum of a substance (see Fig. 15-1). An oximeter is an instrument that measures the amount of light transmitted through, or reflected from, a sample of blood at two or more specific wavelengths.592 Thus, oximetry is a light measurement (photometric) technique that uses two or more specific wavelengths of the light spectrum to differentiate oxygenated from unoxygenated hemoglobin and to quantitate their relative concentrations. In other words, an oximeter is a dedicated spectrophotometer that is designed specifically to measure oxygen saturation (SO2). Because hemoglobin is a colored substance, it absorbs some of the light that is passed through a blood sample. Furthermore, according to the Lambert-Beer law, the amount of light absorbed at a particular wavelength (i.e., optical density) depends on the concentration of hemoglobin present.591 Similarly, the amount of light transmitted through the blood sample at a given wavelength is related inversely to the amount of light absorbed. Each form of hemoglobin (e.g., HbO2, Hb, HbCO, metHb) has its own unique absorption/transmission spectrum (see Fig. 15-1). The SaO2 level can be measured because oxyhemoglobin and desaturated hemoglobin absorb light equally at some wavelengths, whereas they absorb light differently at other wavelengths. For example, at a wavelength of 805 nm in the near infrared region, oxyhemoglobin and desaturated hemoglobin have identical light absorption properties (Fig. 15-4).593 When two substances absorb light equally at a given wavelength, an isobestic point is said to exist.594 On the other hand, at a wavelength of 650 nm in the red region of the spectrum, there is a large difference in light absorption properties between oxyhemoglobin and desaturated hemoglobin (see Fig. 15-4). The total hemoglobin can be determined at 805 nm and the amount of HbO2 can be found at 650 nm. Thus, the difference in light absorption at these two wavelengths can be used to calculate SaO2. Because the presence of cells in the blood tends to scatter light, measurements of SaO2 by oximetry in the laboratory are made usually after breaking down the red blood cells (i.e., hemolysis). Typically, red blood cells are hemolyzed ultrasonically within the oximeter to make the sample more homogeneous and to increase the accuracy of the measurement. Transmission oximeters that use hemolyzed blood are generally accurate, stable, and precise.595 Figure 15-5 illustrates the location of the major components used in transmission oximetry compared with backscatter (reflection) oximetry. Multiple (two) wavelength oximeters are shown in both examples that use red and infrared light sources. The major difference in the two techniques is simply the location of the photodetector. In transmission oximetry, the photo detector is opposite the light source, whereas in reflection oximetry it is on the same side as the light source. Regarding interpretation of data, it is important to understand two essential points when SaO2 is measured using the two-wavelength method. First, when only two wavelengths are used, concentrations of abnormal forms of hemoglobin (e.g., HbCO, metHb) cannot be detected.594 Second, the SaO2 value measured in this way is the percentage of HbO2 compared with the sum of HbO2 and desaturated Hb only (however, HbCO and generally metHb will be picked up by the oximeter as HbO2). Because this measurement does not include abnormal forms of hemoglobin, it is sometimes referred to as functional SaO2.594 Functional SaO2 is the percentage of HbO2 compared with the quantity of hemoglobin capable of carrying oxygen. Functional SaO2 is in contrast with the SaO2 measurement resulting from use of a COoximeter (i.e., cuvette oximeter).591 As the name implies, this instrument can measure HbCO% in addition to SaO2. In addition, the percentage of methemoglobin is usually measured as well. With this instrument, major dyshemoglobin species are included in the determination of total hemoglobin and therefore the calculation of saturation.596 The clinician should be aware that a potential error may occur when CO-oximetry is used in neonatal/premature infant SaO2 assessment. Erroneously high HbCO% and erroneously low SaO2 levels may be reported if substantial quantities of fetal hemoglobin are present. The error is introduced because the absorption properties of fetal oxyhemoglobin are similar to those of HbCO at the light wavelengths used.597 As early as 1935, Matthes showed how transmission oximetry could be applied to the external ear.598 Throughout the years, however, the major problem with noninvasive oximetry has been the inability to differentiate light absorption due to arterial blood from that due to all other blood and tissues in the light path. Two techniques were developed in an attempt to isolate arterial blood and to get a more accurate SaO2 reading. First, attempts were made to arterialize the ear by enhancing local perfusion. Arterialization could be accomplished by one or more of the following: heating the ear, applying a chemical vasodilator (e.g., nicotine cream),599 or briskly rubbing the ear for about 15 seconds. In 1976, Hewlett-Packard incorporated these principles into the development of the model 47201A ear oximeter. This device used the aforementioned principles and measured light transmission at eight different equally spaced wavelengths from 650 to 1050 nm. Measurements at all eight wavelengths were incorporated into a complex formula that corrected for light absorption due to skin pigmentation and provided a measure of functional saturation. This clinical instrument was accurate over a saturation range of 65% to 100%.600 The original Hewlett-Packard ear oximeter was used widely in pulmonary function laboratories, cardiac catheterization laboratories, and physiologic research. Furthermore, the fact that ear oximetry could measure oxygen saturation under both stable and rapidly changing conditions rendered it a very useful diagnostic tool.601 Simple ear oximetry has not, however, proved accurate enough to be used for determining the appropriate oxygen prescription for patients requiring supplemental oxygen during exercise.602 Ear oximetry has never achieved prominence as a clinical bedside monitor because of its bulky nature and relatively high cost. The Hewlett-Packard ear oximeter is no longer being manufactured.603 Pulse oximeters are typically being used in its place. The simplicity and ready availability of pulse oximetry has literally revolutionized clinical oxygenation monitoring. Currently, blood oxygenation can easily be monitored continuously and noninvasively at the bedside or in the office or home. Application of this technology requires minimal technical skill and knowledge regarding the assembly, application, and maintenance of equipment. Pulse oximeters are typically calibrated at the factory and undergo a self-diagnostic check when powered up.628 Furthermore, arterial blood gases with related risks, complications, and costs can often be avoided by using pulse oximetry.289 Indeed, pulse oximetry is currently a “standard of care” in the operating room and is probably soon to become a “standard of care” in critical care and other healthcare settings. It has already been referred to as a standard of care for nearly all patients in neonatal and pediatric intensive care.628 No other medical device has achieved such widespread acceptance and implementation.610 A MEDLINE (National Library of Medicine) search of the term pulse oximetry in 2003 yielded more than 2300 citations,628 and the list is growing rapidly. Furthermore, pulse oximetry has been demonstrated to be the single most important identifier of critical mishap events.611 Capnography is second, with ECG a distant third. The value of pulse oximetry is such that it has often been referred to as a fifth vital sign.612 628 Although photoelectric plethysmography (to be described in the next section) and spectrophotometry have been available for decades, not until 1972 did the Japanese biochemical engineer Takuo Aoyagi591 successfully combine these techniques in the development of the pulse oximeter. Also, the development of microprocessors and LEDs paved the way for clinical pulse oximetry by providing lightweight, stable light sources and bedside computerization of complex mathematical formulas. The Japanese firm Nihon Kohden developed Aoyagi’s instrument and received a Japanese patent in 1974.615 By 1988, the number of companies that sold pulse oximeters under their own brand names increased to 29; 45 different oximeter models were available.607 This relatively new technology had grown exponentially in just a few years. Furthermore, based on the expanding applications of pulse oximetry, it appears that this trend is likely to continue. A plethysmograph is a device for measuring and recording changes in volume of a part of the body or an organ. Photoelectric plethysmography, originally described in 1937,604 is a technology that makes use of light transmission properties to detect the changes from one moment to another in blood volume that occur in a finger or toe. These changes are presumably due to the pulsating arterial vascular bed. Thus, this technology may be used to detect the presence or magnitude of a pulse.605 As you may recall from the Lambert-Beer formula, the pathlength in an absorbing medium affects the amount of light absorption/transmission at a given wavelength. Simply, decreased light passes through the medium as the thickness (volume) increases (Fig. 15-6). If a vascular bed (e.g., finger) is positioned between a light source and a photo detector, pulsatile blood flow can be detected because the amount of light absorbed is in proportion to the volume of blood present (Fig. 15-7). The pulse oximetry sensors can be placed on a variety of sites as shown in Fig. 15-8. The graphic representation of the pulse can also be displayed and is known as a plethysmogram (Fig. 15-9). Constant (static) light absorption occurs due to tissue and venous blood, whereas variable (dynamic) absorption occurs due to pulsatile arterial blood. Photoelectric plethysmography has been used to monitor the hemodynamic status of patients after surgery. In general, when blood pressure or local blood flow is high, the pulse amplitude is high. Conversely, in the presence of vasoconstriction or hypotension, pulse amplitude decreases (Fig. 15-10). Changes in the plethysmogram may indicate the onset of hemodynamic problems and may suggest the need for prompt intervention. More important, detection of the pulse allows for the noninvasive determination of oxygen saturation. In pulse oximetry, baseline absorption is the amount of light that is absorbed during diastole in the measured pulse cycle. The availability of the pulse allows light absorption due to tissue, bone, and venous blood to be canceled out. In addition, any ambient light that reaches the photo detector is likely canceled out. Thus, detection of the pulse and diastole allows us to zero out constant sources of interference and to calculate baseline absorption. Changes in light absorption during systole can therefore be presumed to be due to the addition of pulsatile arterial blood in the light path (see Fig. 15-9).608 The mere functioning of a pulse oximeter, however, should not be interpreted as evidence of adequate perfusion or tissue oxygenation.606 It is also wise to question pulse oximetry readings when the heart rate of the oximeter differs greatly from other indicators and measurements of heart rate. Newer pulse oximeter designs (e.g., signal extraction technology [SET]) actually calculate oxygen saturation as measured with pulse oximetry (SpO2) through complex algorithms without first referencing the pulse rate.629 The schematic illustration of two-wavelength transmission oximetry shown in Figure 15-5 is closely parallel to the structure and function of most pulse oximeters. On one side of the finger are two LEDs that transmit light alternately through the tissue to the photodetector (light detector) on the other side. Both the LEDs and the photodetector are aligned directly opposite each other and are encased within the probe. One LED emits light at a wavelength of 660 nm in the red range, whereas the other LED emits light at 940 nm in the infrared range.608 These two wavelengths are used because they facilitate differentiation of oxyhemoglobin from deoxygenated hemoglobin (Fig. 15-11) and calculation of saturation. At 660 nm in the red range, light absorption of deoxygenated hemoglobin is 10 times higher than light absorption by oxygenated hemoglobin. However, at a wavelength of 940 nm in the infrared range, light absorption by oxygenated hemoglobin is substantially higher than light absorption by deoxygenated hemoglobin. Thus, saturation can be computed through the ratio of light absorption changes (red/infrared) that occurs during systole (see Fig. 15-9; Fig. 15-12). The photodetector actually measures light during three modes: the red light mode, the infrared light mode, and when both lights are off.616 630 The third mode (i.e., when both lights are out) helps insure that light (or noise) from any other source that may be reaching the detector is also canceled out. Photodetectors may sample light as frequently as 480 times per second.616 Although technology continues to improve, a reading should be attainable within at least 2 minutes.617 The response time to actual arterial oxygenation changes also depends on the location of the probe.630 Probes placed on the ear respond quickest whereas finger probes may take up to 12 seconds longer. Probes placed on the toes show an even greater lag time for response. In summary, pulse oximeters are two-wavelength oximeters that measure light transmission both before and during a pulse by incorporating the principles of photoelectric plethysmography.609 The difference in transmission at both wavelengths during a pulse provides a measure of blood oxygen saturation. If a pulse cannot be detected with traditional technology, oxygen saturation cannot be measured. The accuracy of pulse oximeters in measuring exact saturation has been shown to be about ±4% as compared to blood oximetry measurements.619 628 There is a general tendency to be less accurate (i.e., ± 6%628) as saturation falls particularly to less than 70%. The readings are most often falsely high at true low readings but some may actually be falsely lower.620 621 This is not particularly surprising because different instruments use different pulse detection and SaO2 calculation algorithms.621 Furthermore, most of these algorithms were developed based on saturations of normal volunteers and therefore correlate better with high or normal saturations. One early study reported in 1991 found that only 10% of pulse oximetry readings in patients with poor perfusion were actually accurate to within ±4%,631 but newer models and technology have improved accuracy substantially. Some guidelines recommend comparing directly measured simultaneous saturations (i.e., co-oximetry readings) with various pulse oximeter readings to get a baseline relationship.622 These same guidelines also recom-mend periodic comparisons as the patient’s condition changes. These comparisons would also help the clinician understand the standard biases of the specific devices being used. Notwithstanding, this is generally not practical or cost-effective. Most importantly, the clinician should understand that arterial blood gases are superior for diagnostic purposes whereas pulse oximetry is most beneficial as a real-time monitoring device. Interestingly, in one study of postoperative open-heart surgery patients, pulse oximetry seemed to cause slight elevations in readings as compared to saturations measured in the blood.623 The authors suggested that perhaps slight elevations in HbCO secondary to hemolysis or infusion of stored blood may have been responsible for the elevated readings. Methemoglobin may likewise alter pulse oximetry readings. The clinician should be mindful that nitrites, benzocaine (local anesthetic), or dapsone (antibiotic used in the treatment of malaria or Pneumocystis carinii infection) may cause serious methemoglobinemia. There is reasonable evidence to suggest that the readings tend to migrate toward 85% (see Chapter 7). Thus, lower than expected pulse oximetry readings would occur when true saturation exceeded 85% whereas higher readings would occur in severe methemoglobinemia. Like carboxyhemoglobinemia, the clinician should always be alert to the potential causes and possibility of methemoglobinemia especially when cyanosis is observed without substantial decreases in SpO2. Like carboxyhemoglobinemia, sampling of blood via CO-oximetry is necessary for confirmation.
Noninvasive Blood Gas Monitoring
OXIMETRY
Historical Development
Spectrophotometry
Qualitative Analysis
Colorimetry
Photoelectric Effect
Quantitative Analysis
Lambert-Beer Law
Optical Density
Oximeters
Transmission Oximetry
Hemolysis
Backscatter Oximetry
Functional Saturation
CO-Oximetry
Ear Oximetry
Background
Hewlett-Packard Ear Oximeter
PULSE OXIMETRY
Overview
Historical Development
Photoelectric Plethysmography
Pulse/Circulation Dependency
Two-Wavelength Methodology
Technical Limitations
Accuracy
Technical Error
Hemoglobin Variants
Stay updated, free articles. Join our Telegram channel
Full access? Get Clinical Tree
Noninvasive Blood Gas Monitoring
Only gold members can continue reading. Log In or Register a > to continue
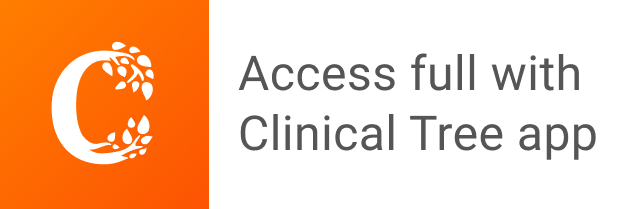