Fig. 9.1
Forced oscillation measurement in a 5-year-old child. Children should be seated upright with their head in the midline and neutral position. The child breathes through a mouthpiece incorporating a bacterial filter with a nose clip in place. Firm support of the cheeks and roof of the mouth is important to minimize the pressure loss of the oscillatory signal
Acceptability and Repeatability of FOT Measurements
Acceptable measurements should be free of artifact including leak, swallowing, mouth movements, talking and other noises and obstruction of the mouthpiece with the child’s tongue. These criteria can be assessed through visual inspection of the Zrs spectra and the individual pressure and flow recordings. In young children additional feedback from the staff member supporting the cheeks and the floor of the mouth can be very helpful. A minimum of three to five acceptable measurements should be obtained and the average and standard deviation (SD) of all acceptable measurements are reported [8, 9]. The most commonly reported FOT outcomes are the Rrs and Xrs at individual specific frequencies and reported as Rrsf and Xrsf (for example Rrs and Xrs at 8 Hz are denoted as Rrs8 and Xrs8), the resonant frequency (Fres) and the area under the reactance curve (AX; defined as the area under the Xrs curve from a defined frequency to the resonant frequency) [18]. Figure 9.2 illustrates these commonly reported outcomes in a healthy child and a child with cystic fibrosis (CF).
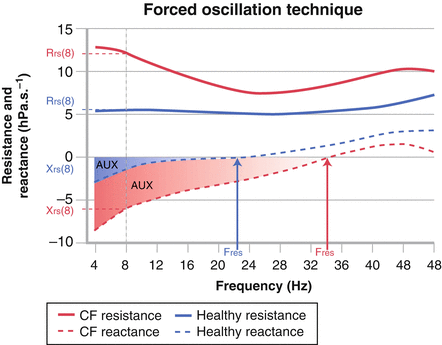
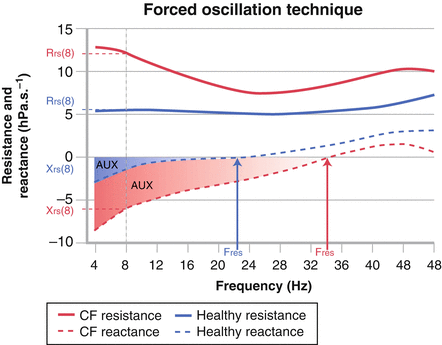
Fig. 9.2
Respiratory impedance spectra from a healthy child (shown in blue) and a child with cystic fibrosis (shown in red) of similar age and heights. The respiratory resistance (Rrs: solid line) tends to be increased and show negative frequency dependence at lower frequencies while respiratory reactance (Xrs: dashed lines) is decreased (more negative) in children with lung disease. Commonly reported FOT outcomes include the Rrs and Xrs at a specific frequency (shown here as Rrs and Xrs at 8 Hz: Rrs8 and Xrs8, respectively). The resonant frequency (Fres) is the frequency at which Xrs is equal to zero and represents the point at which the respiratory system recoil (or compliance) and inertance (reflecting the properties of the central airways) are balance. The area under the reactance curve (AUX) is the sum of the Xrs curve from the lowest frequency measured to the resonant frequency
To date there is insufficient evidence to allow the definition of intra-test repeatability that can be used to state that a test session is repeatable. The mean within-test coefficient of variability (CV: defined as the standard deviation (SD)/mean and expressed as a percentage) has been reported as ranging between 5 and 10 % for Rrs and up to 20 % for Xrs [19–22]. Until such time as definitive criteria for repeatability are available users should retain all acceptable measurements and exercise caution when deciding to exclude apparently acceptable data based on repeatability criteria alone.
The between test (inter-test) coefficient of repeatability (twice the SD of the difference between two measurements) in healthy children ranges between 1.1 and 2.6 hPa s/L for Rrs and equates to a relative change of 12–30 % [23–25] with similar short- and long term repeatability reported in children with lung disease [19, 20, 26]. The repeatability of Xrs is reported as absolute value due to the proximity of Xrs values to zero and ranges from 1.2 to 2.0 hPa s/L [19, 20, 23–26].
Reference Ranges in Preschool and School Aged Children Using Forced Oscillations
There is a range of reference equations available for use in preschool and school aged children and to date the majority of these studies are in Caucasian children [24, 27–29] although reference data in children from Mexico [30], Iran [31], Korea [32], and Vietnam [33] are now available. It is not clear what impact ethnicity will have on reported FOT outcomes and this area deserves attention. Not all studies reporting reference equations have reported all of the FOT outcomes outlined above. It is important to note that the frequency range of the FOT outcomes reported by the CareFusion IOS is different to that of the Cosmed Quark I2M and as such reference equations are currently equipment specific. Users should carefully review the available studies for suitability relating to local equipment and patient populations.
The Role of the Forced Oscillation Technique in Clinical Practice
The use of the FOT in the management of individual children with lung disease remains unclear with only limited information on its potential use. The primary reasons for this shortage of studies assessing the clinical role of the FOT are likely to be the historical lack of standardized methodological guidelines and availability of commercial equipment. The clinical utility of the FOT in young children with recurrent wheeze and/or asthma, CF and in those children born preterm with or without bronchopulmonary dysplasia (BPD) has recently been comprehensively reviewed as part of an ATS workshop report on optimal lung function tests in young children [34], while the role of the FOT in older children with lung disease remains to be formally assessed.
The change in FOT outcomes following bronchodilator inhalation considered to be clinically relevant is reasonably consistent across multiple studies. A clinically relevant bronchodilator response is defined using the 5th/95th centiles of the response to bronchodilators in healthy populations. These have been reported to be between −33 and −42 % for Rrs, 61 and 70 % for Xrs and approximately 80 % for AX, irrespective of the dose of the salbutamol [25, 27, 33, 35–37]. The ability of the FOT to assist in the diagnosis and/or management of asthmatic and/or wheezy children either before or after bronchodilator inhalation remains unclear. Some studies demonstrated no differences in the baseline lung function or bronchodilator responsiveness between healthy and asthmatic children [22, 35–37]. In contrast, other studies have reported that FOT may provide some benefit in the identification of children with asthma [38–41]. One study from Shi et al. [42] suggest that FOT outcomes may predict loss of asthma control and therefore may have a role in the management of children with asthma and further work in this area is needed.
The FOT has been used to assess lung function in children with CF; however, the majority of these studies have been cross-sectional with only limited assessment of pulmonary infection or inflammation and therefore the ability of FOT to assist in the clinical management of younger children with CF is unclear. Brennan et al. assessed the relationship of respiratory mechanics derived from the low-frequency FOT [43] in a group of infants and young children with CF at the time of an annual bronchoalveolar lavage and reported increased pulmonary inflammation, but not infection, was associated with increased respiratory resistance. However, this variation of FOT is not easily transferred to a clinical setting. Most studies using commercially available FOT equipment suggest that the FOT is not sensitive to the early CF lung disease either cross-sectionally or longitudinally [26, 44–48]. In contrast, increased Rrs and decreased Xrs have been reported in young children with CF in the presence of respiratory symptoms [19].
The primary impact of preterm birth and BPD on the respiratory system is likely to be the peripheral lung with altered alveolar structure [49]. As such the FOT should be a particularly suitable test for use in infants and children following preterm birth, particularly those born very preterm (<32 weeks gestational age). Despite this the FOT has not been used widely in infants and children born preterm. Using the low-frequency FOT Pillow et al. demonstrated that oscillatory mechanics can be measured in preterm infants around term [3]. Studies using commercially available equipment have demonstrated that FOT outcomes are abnormal in both preschool and school aged preterm children, both with and without BPD and that these differences are more pronounced in measures of respiratory reactance than resistance suggesting that the FOT is sensitive to the altered peripheral lung pathophysiology evident in these children [20, 50, 51]. There are limited reports of the FOT being applied in children with upper airway dysfunction and further research in this patient group is required [52–54].
Future Work and Conclusions
The measurement of respiratory system impedance has the potential to provide a great deal of information on a variety of conditions during the early years of life; however, further work is required if the FOT is yet to reach its full clinical potential. In the short term it is important to identify which FOT outcomes are most sensitive to each specific pediatric lung disease with the knowledge that the pathophysiological mechanisms of many respiratory diseases exhibit strong peripheral lung involvement during early life. In the longer term it is important to gain an understanding of how respiratory mechanics alters longitudinally during development and what kind of deviation from this path requires intervention.
The Multiple Breath Washout Technique
Inert gas washout was first described over 60 years ago following the advent of fast responding gas analysers [55, 56], but it was not until the development of personal computers that breath-by-breath analysis became feasible and enabled MBW analysis techniques as we know them today. The choice of a suitable inert gas for MBW testing should consider if the inert tracer gas is safe for patients to inhale and does not participate in gas exchange or dissolve significantly in the blood or other tissues. Inert gases may either be resident within the lung (i.e., present within room air, e.g., Nitrogen (N2) or Argon) or non-resident (e.g., Sulfur hexafluoride, SF6, or Helium).
Inert gas washout tests allow the distribution of ventilation to be assessed. Ventilation within the lung is determined by the structure of the respiratory airway tree and the gas exchange unit or alveoli. Gas transport and mixing by convection (i.e., bulk flow) predominates in the conducting airways. In the lung periphery, bulk flow is minimal and gas transport by molecular diffusion dominates. In the region of the entry of the acinus, the relative contributions of convection and diffusion to gas mixing are equal, generating a “diffusion-convection front” in the healthy adult lung [57]. Pathological processes affecting the dimensions of these peripheral airways in a heterogeneous, or patchy, manner, affect the distribution of ventilation. It is this unevenness of ventilation that is detected by tests such as the MBW technique. A typical MBW test is performed over a series of tidal breaths, requiring minimal cooperation or coordination, offering feasibility across the entire pediatric age range. Improved sensitivity to detect lung disease, in comparison to conventional spirometry, across a number of important pediatric lung diseases [58–61].
The Technique Methodology
The MBW examines the pattern by which an inert gas is washed out of the lungs during tidal breathing. Standardization guidelines for equipment validation, MBW test performance and subsequent analysis have recently been developed for use in all age groups and are covered in more detail elsewhere [62]. Inert gas washout is based on accurate measurement of respiratory flow and inert gas concentration signals, which need to be correctly aligned in time prior to subsequent analyses. The majority of equipment in publications prior to the early to mid-2000s has been custom-made and based on a variety of flow measurement devices and inert gas analysers. In recent years commercial systems have been developed, based on SF6 measurement for the infant age range (Exhalyzer D, ECO Medics AG, Switzerland) or on N2 measurement suitable for preschoolers and above (Exhalyzer D, ECO Medics AG, Switzerland; Easyone Pro, ndd Medical Technologies, Switzerland). Recent advances in equipment validation [63] appear to have provided robust clinical devices suitable for widespread use.
For the patient, minimal cooperation and coordination are required: an adequate mouthpiece/facemask seal must be maintained to prevent leaks, and a regular breathing pattern needs to be maintained. A facemask is used in infants and may be used for preschool children due to difficulty maintaining an adequate mouthpiece seal. A regular breathing pattern is achieved in infants by performing the test during natural sleep or under sedation (in the supine position), or in older children, sitting upright and using distraction with an interesting video [62].
In N2-based MBW the nitrogen is washed out of the lung by switching the patient into breathing 100 % O2. Non-resident inert gases require an additional wash-in phase during which the inhaled inert gas concentration (typically 4 % SF6 or 4 % He with 21 % O2 and the balance N2) equilibrates within the lung, before being washed out by breathing room air. Initial MBW studies demonstrated feasibility using N2 as the inert gas [64–66], but inhalation of pure O2 in infants was subsequently shown to alter normal tidal breathing patterns [67], with potential effects on subsequent calculated indices. As a result interest in non-resident inert marker gases increased, with strong feasibility subsequently demonstrated for SF6-based MBW [58, 68–70]. The clinical utility of the MBW may be hampered by the test duration which may be up to an hour in some children. Recent efforts to shorten the MBW protocol suggest the test duration can be shortened [71, 72]. Feasibility within the busy clinical environment and in more remote settings has also recently been demonstrated [73, 74].
Available MBW Indices
Each MBW test is conventionally performed until the end-tidal inert gas concentration reaches 1/40 of its starting concentration (i.e., approximately 2 % for N2 MBW and 0.1 % for SF6/He MBW at the concentrations mentioned prior) (Fig. 9.3). Three technically acceptable tests should be the target of each testing session. In addition to providing information about ventilation distribution, lung volume data (functional residual capacity, FRC), trapped gas and measures of the volume of the conducting airways (Fowler [75] and Langley [76] airways dead space) can also be determined. These ventilation inhomogeneity parameters can be grouped into those reflecting the presence of overall global abnormalities, such as the Lung Clearance Index (LCI) [77] and moment ratios [78], and those based on more sophisticated phase III slope analysis providing additional information about the location of any abnormality [79].
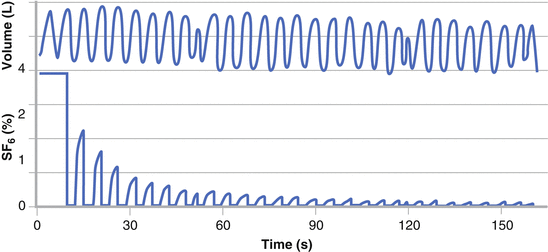
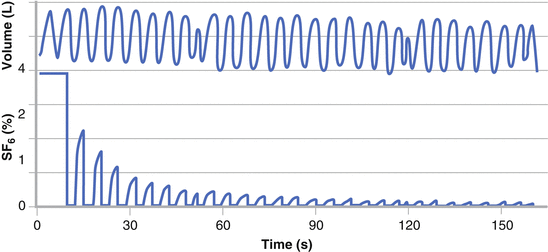
Fig. 9.3
Real time tracings from a MBW test. Tidal volume (upper panel) and inert gas SF6 concentration (lower panel) are displayed. The washout phase of the MBW test commences after equilibration of SF6 concentration within the lungs to approximately 4 % and continues until SF6 concentration has decreased to below 1/40th or 0.1 %
LCI is the simplest parameter to calculate, the easiest for physicians and patients/parents to understand conceptually, and the most popular index reported in the recent pediatric literature. It represents the number of lung volume turnovers (TO, or FRCs) required to clear the lungs of the inert marker gas to 1/40 of the starting concentration. Moment ratios (calculated using an approach termed “moment analysis”) are more complicated and describe the degree of skewing of the washout curve, with increased skewing representing increased release of inert gas at a later stage of the washout [78]. Calculation of moment ratios are described in more detail elsewhere [80]. The proposed advantage of moment analysis is that it may compensate for the impact of variation in respiratory rate and tidal volume during the washout [65, 81].
Phase III slope analysis from MBW is the main index reported in the adult literature, and has been developed from theoretical [79], experimental [82, 83], and adult lung modelling data [83–85], to separate ventilation inhomogeneity arising from conductive airway zones (termed convection-dependent inhomogeneity or CDI) and within more distal acinar zones (where diffusion and convection interact to generate inhomogeneity, termed diffusion-convection-dependent inhomogeneity or DCDI). The CDI and DCDI can be expressed as the clinical indices, S cond and S acin, respectively [86]. The original method for calculation proposed in adults required a strict breathing protocol (tidal volumes 1.0–1.3 L and breathing rate of 10–12 breaths/min), to try to avoid variation in pre-inspiratory lung volume, inspired and expired volumes and flow [57, 87–94] affecting the magnitude of the phase III slope. Adaptation of this method for pediatric testing, where a structured breathing protocol is not feasible ≤16 years, has required the incorporation of a tidal volume compensation for phase III slope values [62]. The use of the strict adult breathing protocol in children has been shown to significantly affect MBW outcomes [45]. Phase III slope parameters do not appear as robust as LCI to variations in breathing pattern, which compromises feasibility to a degree in the preschool age range, whilst the low parenchymal-to-airway volume ratio encountered in infants makes identification and accurate assessment of a phase III slope from tidal breath expirograms challenging. These indices remain exploratory in the pediatric range at present and have not demonstrated the same degree of clinical utility to date as LCI.
Acceptability and Repeatability of MBW Measurements
The majority of the data presented in this section is based on SF6-based MBW, whereas the recent availability of commercial N2-based equipment is starting to generate equivalent data. Strong feasibility exists across all age ranges: in infants 76–90 % during natural sleep (aged <6 weeks) [95, 96] and 87 % in sedated infants [70], compared to 80 % in preschoolers (ranging from 50 % of 2–3-year-olds to 87 % of 5–6-year-olds) [58]. Within-session variability ranges from 3 to 8 % across studies in preschoolers and above, with between-session variability approximately 5 % [97], equating to a statistically significant change of ±1 TO for LCI. A collaborative multiple center approach has led to publication of recent SF6 mass spectrometer based normative data for the entire pediatric age range [98]. Recently preliminary data describing normative N2-based LCI data has also been published from 7 to 70 years of age [99]. Both these sets of normative data illustrate the higher LCI values encountered at the extremes of age (e.g., infancy) but show largely stable values in between, making LCI an attractive longitudinal tool.
The Role of LCI in Clinical Practice
LCI is now strongly supported as a research tool in clinical studies in CF, due to a large body of evidence supporting potential clinical utility in this disease group [51]. The heterogeneous distribution of CF lung disease has been nicely illustrated by recent imaging studies [100], and includes the peripheral airways [101].
Abnormal LCI values are frequently present in CF subjects, from infancy onwards, and disparity between health and disease appears to increase over time, based on this cross-sectional data. Improved sensitivity of LCI to detect lung disease despite normal spirometry exists across a number of studies from the preschool age range onwards [58, 60, 69, 102]. Strong correlation between LCI and high resolution computed tomography (HRCT) measured structural lung damage has also been described in several studies, from infancy [103–106]. Prognostic value is also starting to emerge, with preschool LCI values predicting those subjects with abnormal spirometry at school age [107]. LCI improves with treatment intervention [108–110], and its potential to detect significant changes despite small cohort sizes is exciting for future intervention studies. However, heterogeneity of response encountered in established disease [80] may limit utility in more severe subjects.
As in CF, the heterogeneous distribution of the disease process in asthma, involving the entire airway tree, not just the central airways, has been demonstrated in imaging and pathology studies [111–113], predicting potential utility for MBW. Pediatric asthmatics have increased global ventilation inhomogeneity (LCI and moment ratio values), compared to controls [65, 114]. Phase III analysis describes a predominant conducting airways pattern of abnormality (elevated S cond) [115]. Improved sensitivity to detect disease involvement, in comparison to spirometry, is suggested by both pathological gas trapping in childhood and adolescent asthmatics [116], and elevated LCI values in well controlled childhood asthmatics [61], despite normal baseline spirometry in both groups. Whether this represents established airway remodelling is unclear. Increased ventilation inhomogeneity has also been suggested as an important mechanism for airway hyper-responsiveness in adult asthmatics [113, 117].
In younger preschool recurrent wheeze phenotypes, MBW also appears to differentiate between multi-trigger wheeze and viral-induced wheeze phenotypes (increased LCI and S cond values) [118]. Establishing a predictive value to detect those who subsequently develop classical asthma will require longitudinal studies. Utility in challenge testing is suggested by the ability to detect a marked peripheral airways response, missed by spirometry, on challenge testing [119], but the time-consuming nature of the test compromises routine clinical utility in this setting. The same applies for assessment of bronchodilator response. Response to treatment with therapy targeting the peripheral airways (e.g., fine particle inhaled corticosteroid) has been demonstrated in adults [120], but corresponding pediatric data is lacking.
Discussion of clinical utility of MBW in infants with BPD illustrates the importance of disease distribution when assessing potential utility. “Old” BPD cross-sectional studies describe elevated gas trapping (as assessed by FRCpleth and FRCN2) and LCI (or moment ratios) in BPD infants during the newborn period, in comparison to healthy controls [81, 121]. However, recent larger multicenter studies, of “new” BPD, show little difference between groups [95, 96]. This probably reflects both improved study design, adequately correcting for important confounders such as prematurity and intrauterine growth, and the more diffuse nature of “new” BPD, generating less marked ventilation unevenness.
Future Work and Conclusions
The availability of robust commercial equipment and improved standardization of the MBW technique represent essential steps if exciting potential utility suggested by research studies to date is to translate into feasibility in the busy clinical laboratory. Strongest utility is currently suggested for CF, but may also exist for asthma and other disease groups. Efforts in the future to shorten test duration are important and evaluation of current commercial equipment is ongoing. Longitudinal studies will provide a clearer idea of true utility.
The Interrupter Technique
The interrupter technique allows the measurement of the resistance of the respiratory system, including the airway tree, lung tissue and chest wall. The primary advantage of the technique is that it requires minimal cooperation from the individual being tested and hence is of particular interest in infants and young children. There have been a number of studies conducted in sedated and unsedated sleeping infants [122–128] and spontaneously breathing children as young as 2 years [24, 129–133]. Historically, there was little standardization of the technique. The availability of commercial equipment and the release of guidelines for its use in preschool children [8] should lead to an improved understanding of its role in the clinical management of young children [34].
The classical description of the interrupter technique involves the rapid occlusion of the airway opening and the measurement of the flow immediately preceding the interruption and the changes in airway opening pressure (Pao) following the interruption. The interrupter resistance (Rint) is derived from the change in Pao by the flow. An alternative approach derives the Rint using the change in Pao and the flow immediately after the interruption [134]. The outcomes derived from these two approaches differ and cannot be used interchangeably [135]. The classical approach is the most commonly used and unless specified otherwise is the technique for which details are provided in this chapter.
The Interrupter Technique Methodology
Commercial equipment for both the classical and the alternative methods of measuring the Rint are available. The interrupter technique assumes that following the interruption to airflow the alveolar pressure rapidly equilibrates with the airway opening and that the change in Pao reflects the pressure drop across the whole airway tree and therefore equates to the alveolar pressure [128, 136, 137]. Following occlusion two distinct phases are seen in the Pao trace (Fig. 9.4). There is an initial rapid rise in Pao that reflects the resistive drop across the airway tree and a component of the resistive properties of the lung and chest wall. This is followed by a slower rise to a plateau and reflects the stress relaxation of the respiratory tissues.
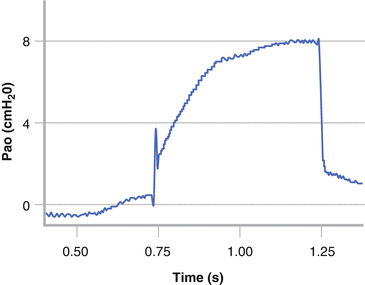
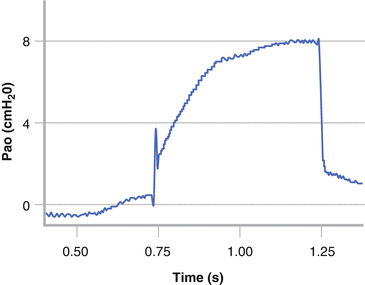
Fig. 9.4
Interrupter resistance (Rint) measurement in an infant. The airway opening pressure (Pao) has an initial rapid, followed by a slower, secondary rise. The pre-occlusion flow and post occlusion Pao are used to derive Rint
The Rint is influenced by the closure time of the occlusion valve [128], the compliance of the upper airways [128], the type of patient interface (face mask or mouthpiece) used [126, 138, 139] and the method of determining the change in Pao following the interruption [126, 140, 141]. The most commonly used approach for the derivation of the Pao is to use the linear back extrapolation of Pao between 30 and 70 ms following the interruption [8].
In infants Rint is measured with the infant in a supine position during quiet sleep. The facemask is placed over the nose and mouth of the infant and supported firmly to minimize pressure loss across the cheeks and floor of the mouth [126]. The use of equipment designed for older children and not adapted for infants is not recommended, with low success rates and poor repeatability of Rint reported [122, 123]. Recommendations for the measurement of Rint in young children are available and in the absence of detailed guidelines in older children should be used for all measurements of interrupter resistance in cooperative children [8]. In preschool and school aged children measurements are made with the child seated and looking directly ahead while breathing through a mouthpiece and with a nose clip in place and the cheeks firmly supported during measurements. The airway is occluded during expiration with the interrupter valve for a period of 100 ms at a flow equating to the peak tidal expiratory flow. A minimum of ten interruptions should be obtained with at least five acceptable measurements retained.
Acceptability and Repeatability of Interrupter Technique Measurements
Measurements should show a smooth increase in airway opening pressure to a peak and be free of leak or unusual changes in pressure and/or flow at the time of the interruption. The median of all acceptable measurements is reported. The within-test repeatability of Rint in healthy infants is dependent on the analysis technique [126] and the mean (or median) coefficient of variation has been reported to range 16.6–19.3 % [124–126] The data available for the between test repeatability of Rint in infants is scarce with Fuchs et al. reporting the mean between test difference in 22 unsedated 6 week old infants to be 1.4 hPa s/L with the limits of agreement being −21.2 and 23.9 hPa s/L [124].
In contrast there are many studies that have assessed the within- and between-test repeatability of Rint in preschool and school aged children and these are extensively reviewed elsewhere [8, 34]. The coefficient of variability of a single testing session ranges between 10 and 12 % of the median Rint value [133, 142–145]. The short term (15–30 min) and longer term (2 weeks to up to 3 months) repeatability of the Rint has been assessed in healthy children and in children with lung disease. The coefficient of repeatability (equating to two times the standard deviation of the measurement) ranges from 1.7 to 4.4 hPa s/L [132, 146, 147].
Reference Ranges for Interrupter Resistance in Infants, Preschool and School Aged Children
Fuchs et al. [124] reported upper limits of normal for Rint in unsedated infants aged 5–7 weeks of age. To date only one study reported reference ranges for the Rint in sedated infants over a broader age range [125]. Measurements of Rint in healthy preschool and school aged children have been reported in Caucasian children [24, 132, 133, 143, 148] as well as children of differing ethnic backgrounds [125, 148–150]. Collated reference values for the classical technique in children aged 3–13 years have been developed and offer the most robust references ranges currently available [151].
The Role of the Interrupter Technique in Clinical Practice
The potential role for measurements of Rint in infants has not been assessed. The lack of appropriate commercially available equipment and standardized methodology guidelines in this age group limit the ability of health professionals and researchers to assess the ability of Rint to assist in the diagnosis and/or management of infants with lung disease.
The clinical role of Rint in preschool children has been extensively reviewed by the ATS workshop report on optimal lung function tests in young children [34]. While studies reporting measurements of Rint in older, school aged children show responsible agreement with other clinically available lung function tests [144] its primary role is likely to be in younger children unable to perform these tests accurately.
The limits of agreement for the determination of a clinically relevant bronchodilator response in Rint obtained with the classical technique are similar and are reported as a decrease in Rint of >2.5 hPa s/L or >32–35 % of baseline [143, 152, 153].
The majority of studies using the interrupter technique have been in children with a history of recurrent wheeze or cough and asthma. The majority of studies have reported that children with asthma have increased Rint compared to healthy controls; however, the proportion of asthmatic children with Rint outside of the normal range varies significantly and these differences are likely to relate to subject selection (community versus hospital/clinic asthmatics) and current asthma treatments [36, 131, 133, 144, 145, 152]. Children with asthma or recurrent wheeze tend to have larger decreases in Rint following bronchodilator inhalation when compared to healthy children, and studies have reported sensitivity and specificity ranges from 24 to 76 % and 70 to 92 %, respectively to distinguish between asthmatic and healthy children [36, 148, 152]. However, each of these studies expressed the bronchodilator responses differently making direct comparisons difficult. A number of investigators have assessed the Rint in placebo controlled clinical trials of asthma medications [131, 154–156] with significant improvements in Rint seen in some [131, 155] but not all trials [154, 156], and provide early evidence that Rint may be a useful clinical trial outcome measure in young children with asthma.
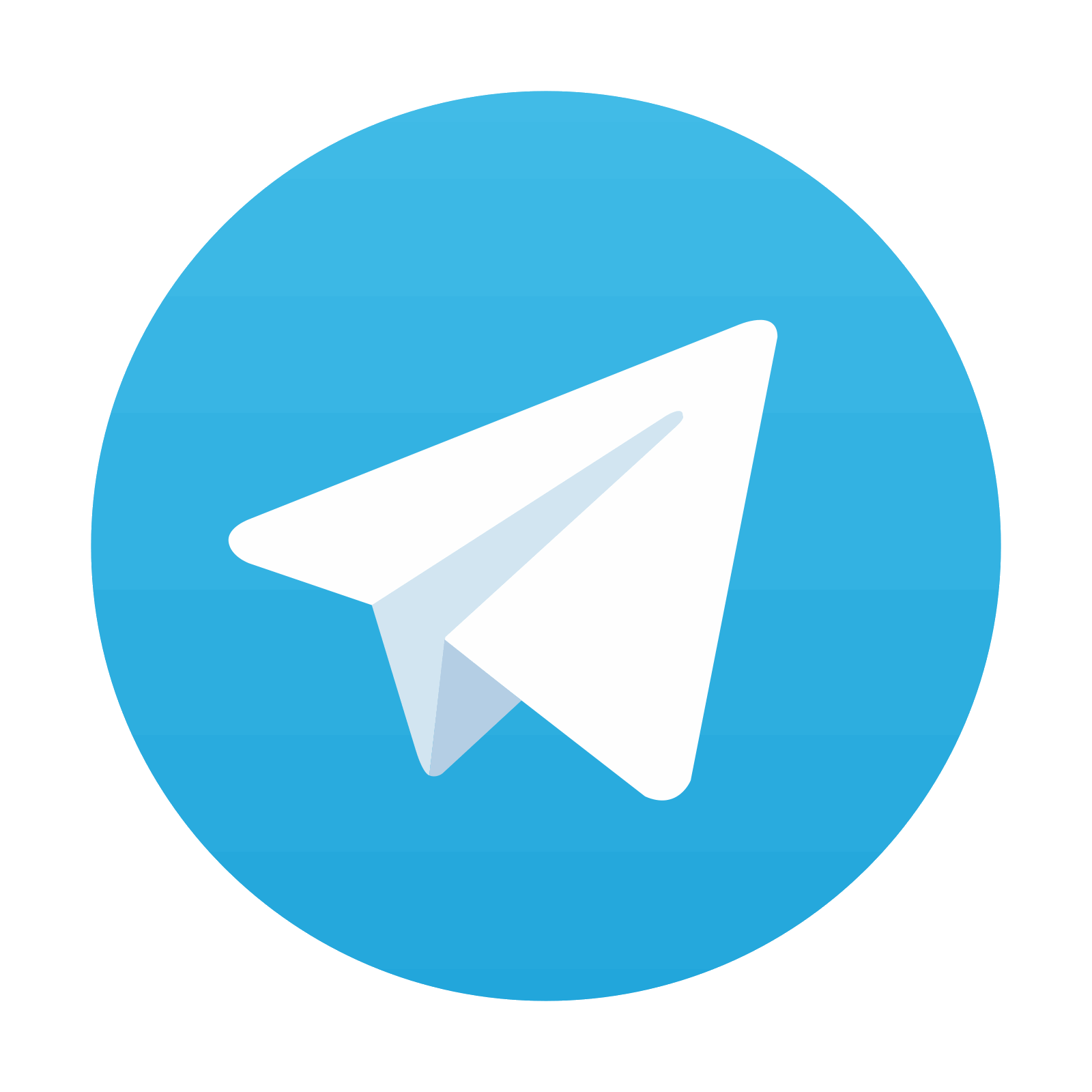
Stay updated, free articles. Join our Telegram channel
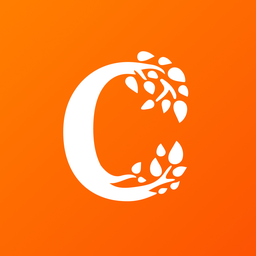
Full access? Get Clinical Tree
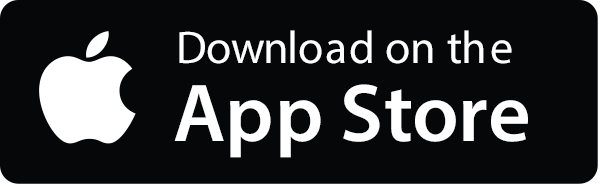
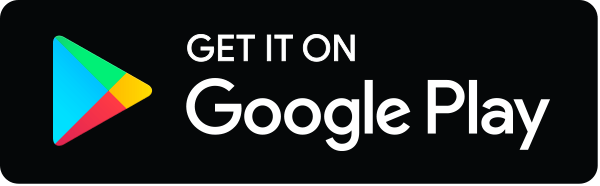