Fig. 4.1
Development of the lung buds in the early chick embryo. At HH stage 17 (day 2.5) the laryngotracheal groove forms in the ventral foregut (F) and deepens over time (K), eventually separating from the gut to form the trachea (S). The trachea bifurcates caudally to produce the bronchi (M, T). The lung buds initially form as swellings in the wall of the foregut (D), which grow outwards over time (I, N, V). FG foregut, LG Laryngotracheal groove, ES esophagus, BR bronchi, LB lung buds. From [11]
The branched network that forms during lung development eventually comprises millions of airways arranged in intricate patterns, maximizing the surface exchange necessary for respiration. The branching pattern of the airway epithelium into the surrounding mesenchyme is complex but remarkably stereotyped, and is generated by three geometrically simple modes of branching (domain branching, planar and orthogonal bifurcation), that occur under the influence of local parameters [14]. The genetic basis of the branching program is still not elucidated, although it has been shown that multiple signaling pathways, including the Wnt, bone morphogenetic protein (Bmp), and fibroblast growth factor (Fgf) pathways, are implicated in its regulation [4, 14].
Shortly after the airway branching process begins, differentiation of the airway smooth muscle (ASM) and cartilage, that will give both the rigidity and elasticity critical for effective air exchange in the lungs, commences. ASM is one of the main targets for lung innervation (see section below) and is first detected at E10.5 in mouse [15, 16] and by week 6 of human development [12]. In E10.5 mouse embryos, ASM differentiation is first detected in the mesenchyme overlying the carina (the point where the trachea splits into the main bronchi). While differentiating during the same time window, ASM and cartilage are initially localized to separate domains, with the cartilage differentiating in a much wider domain along the length of the trachea and main bronchi [16]. Recent studies show that, despite previous assumptions, stalk mesenchyme of the epithelial bud does not contribute to ASM, but that ASM progenitors are located in a niche just ahead of branch tips [17, 18]. These cells subsequently migrate along the tip to reach the stalk and begin differentiating into ASM [18]. By E11.5 in the mouse, the ASM domain expands proximally along the trachea and distally along the main bronchi. At later stages, ASM and cartilage develop a complementary spatial pattern of differentiation. Interestingly, a loss of either ASM (by conditional deletion of the smooth muscle differentiation gene Srf) or cartilage (by conditional deletion of the cartilage differentiation gene Sox9) results in an increase in cell number and in the domain of the remaining tissue lineage, suggesting a communication feedback mechanism between ASM and cartilage [16].
Development of Lung Innervation
During the early development of the lungs, a complex neuronal network is laid out. The neurons that contribute to this network are generally divided into two categories: extrinsic neurons, which have their cell bodies outside the respiratory tract (i.e., parasympathetic innervation from the nodose and jugular ganglia in the brain stem, and sympathetic innervation from dorsal root and thoracic ganglia), and the intrinsic innervation composed of local parasympathetic ganglia, as well as true intrinsic ganglia [15, 19–21]. This nerve network is formed from an intricate combination of migrating neural crest cells that differentiate into neurons, and extrinsic axonal outgrowths, the true complexity of which is yet to be fully elucidated. As outlined below, intrinsic neurons have been implicated in important roles in early stages of lung development , whereas extrinsic nerves represent the main regulatory neural influence at later stages, in particular, after birth.
Development of Intrinsic Lung Innervation
Intrinsic lung neurons, whose cell bodies reside within the lungs, are derived from neural crest cells (NCC), a highly migratory, transitory cell population that gives rise to a wide diversity of cell types throughout the embryo including all neurons and glia of the peripheral nervous system [22]. Their development begins with the migration of NCC from the dorsal aspect of the neural tube into and along the embryonic foregut. Fate mapping studies in chick embryos demonstrated that NCC arising in the vagal (hindbrain) region of the neural tube, adjacent to somites 1–7, migrate in a rostrocaudal direction along the entire length of the gut to form the enteric nervous system (ENS), the intrinsic innervation of the gastrointestinal tract [23], and reviewed in [24–26]. As these vagal NCC migrate along the foregut, a subpopulation of cells moves tangentially from the foregut into the adjacent lung buds, a process revealed by NCC tracing in the chick [11] and mouse [19], and by antibody labelling of NCC in the human embryo [12]. This subpopulation of NCC therefore appears to undergo a two-step migration from one organ to another: first from the vagal region of the neural tube into and along the gut, and then tangentially from the (fore)gut into the lungs (Fig. 4.2). Concomitant with this early vagal NCC migration, the trachea and lung buds are in the process of separating from the foregut, so the lung buds and gut endoderm are physically connected, facilitating the direct migration of NCC into the lung buds. At these early stages of lung bud development, NCC are present in relatively high numbers compared to the size of the buds (Fig. 4.2f–i). However, this appears to be a transitory situation, as the lung buds subsequently increase in size dramatically, decreasing this ratio.
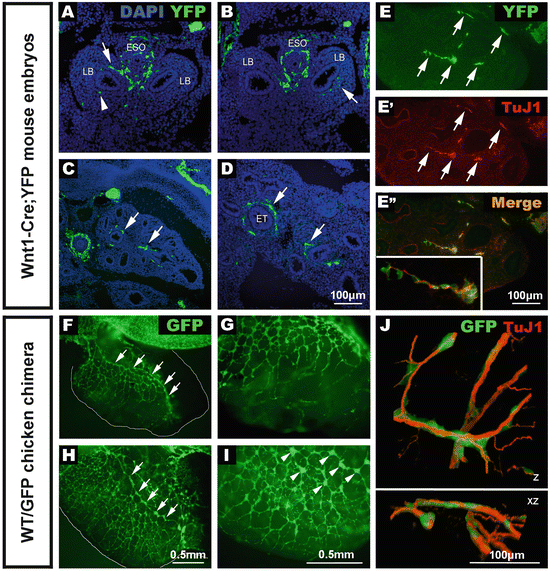
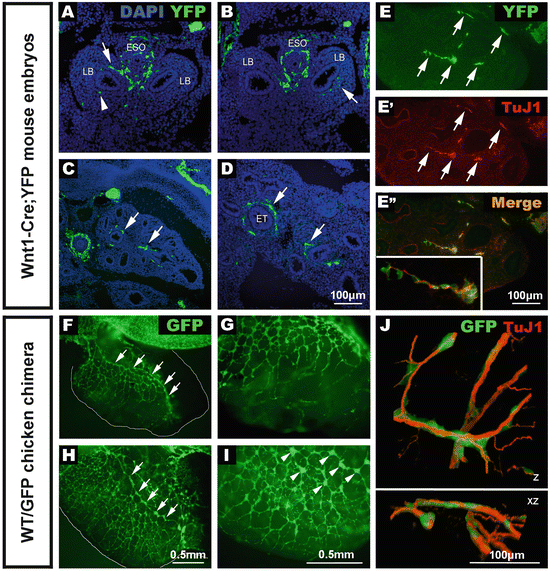
Fig. 4.2
Early development of lung innervation from neural crest cells and extrinsic nerve fibers. (a–e) Sections of Wnt1–Cre; YFP embryos immunostained using anti-GFP. (a, b) E10.5 embryo sections showing positively labelled neural crest cells within the esophagus, trachea , and lung buds. (c, d) E13.5 embryo sections showing numerous YFP cells (arrows) within the developing lungs closely associated with the epithelial tubules. (e) The majority of YFP+ tissue (e) is positive for the neural marker, TuJ1 (e’) and clearly overlaps (e” and inset). (f–i) Wholemount preparations of foregut and lungs from chicken embryos that received a vagal GFP graft to specifically label neural crest cells. (f, g) At E7.5 the network of GFP+ NCC within the lungs is extensive, and an interconnected chain of GFP+ cells runs along the length of the lung (f, arrows), with progressively finer GFP+ projections extending towards the periphery (g). At E8.5 (h, i), numerous GFP+ cells and interconnected ganglia (h, arrows) are present within the lung. High magnification reveals interconnected GFP+ ganglia (i, arrowheads). (j) Association of migrating neural crest cells and extrinsic innervation during chicken lung development . Confocal 3D reconstruction of the developing chick lung at E6, showing the close association of the migrating lung neural crest cells (green) with the extrinsic nerve fibers (red). Z: 3D reconstruction of high magnification confocal image stack. XZ: 90° rotation of the same 3D reconstruction around the X axis. ESO esophagus, LB lung bud, ET epithelial tubule. From [19, 27, 28]
Within the chick lung , vagal NCC have been shown to differentiate to form neurons that are present adjacent to the future airways and are closely associated with ASM [11]. These intrinsic lung neurons appear to aggregate and form the airway parasympathetic ganglia. Lung innervation studies in human [12, 29], pig [30] and mouse [15, 20] demonstrated that intrinsic lung innervation is closely associated with ASM in the distal lung. Surrounding the future airways, a neuronal plexus lies on top of the ASM and extends into the distal lung over time along the growing airways [15]. Intrinsic airway ganglia are often found at conducting airway branching points and act as sites of convergence for several different classes of pulmonary innervation [31]. They receive input from extrinsic and other intrinsic neurons, and may act as sites of signal integration before signaling to downstream targets of innervation in the ASM [32].
Although the mechanisms and signaling cues that direct the tangential migration of NCC from the gut into the lungs have not yet been elucidated, perhaps the most plausible explanation for this behavior is a cell autonomous (chemotactic) response to a lung -specific signal. Such mechanisms, involving the guidance proteins Netrins (netrin-1 and -3) and netrin receptors (DCC, deleted in colorectal cancer, and the adenosine A2b receptor) have been reported to be involved in the perpendicular “secondary” migration of NCC from the outer (myenteric) to the inner (submucosal) layers of the gut and also, interestingly, out of the foregut into the developing pancreas [33], where NCC also form ganglia [34]. However, to date no specific molecular signature, or unique expression of cell surface receptors, has been identified in the NCC that colonize the lung. In fact, in chick embryo studies addressing this question, lung NCC were found to express many genes in common with enteric NCC, including Sox10, EdnrB, and Ret [11]. A large body of evidence has demonstrated the importance of the RET signaling pathway in ENS development , and activation of Ret receptor and Gfra1 co-receptor on migrating enteric NCC by the GDNF ligand is essential for NCC migration, survival, proliferation, and differentiation (reviewed in [35]). Due to this key role for RET in NCC development, and that Ret −/− mice have a depressed ventilatory response to inhaled CO2 [36] the potential role of RET signaling in the development of lung innervation has been investigated by a number of groups. Our group showed that Ret-expressing lung NCC are attracted to GDNF in cultured lungs [19]. Further, studies by Langsdorf et al. [20] demonstrated that in Ret −/− mice the number of intrinsic lung neurons is reduced by 80 % in the trachea and primary bronchi, but these effects were not evident in Gdnf −/− and NRTN −/− mice suggesting redundancy in signaling through the Gdnf family ligands, Gdnf, NRTN, artemin and persephin [37]. However, in conflicting studies, when Freem et al. examined the lungs of Ret −/− and Gfra1 −/− mice, no major differences in the extent of lung innervation were observed [19]. Nevertheless, Langsdorf et al. reported that in Ret mutant embryos intrinsic lung ganglia were reduced in size, further pointing to a possible role for the RET signaling pathway in survival and/or proliferation of lung NCC [20]. Thus although some studies have implicated signal(s) such as Ret in NCC colonization of the lung, other studies raise alternate possibilities. Freem et al. demonstrated that NCC following the enteric migration route along the gut can equally re-migrate to the lungs after back-transplantation from the gut to their initial site of migration within the vagal neural tube [27]. This suggests that vagal NCC are not prespecified to colonize either the gut or the lung and therefore they may migrate into the lung passively or by responding to (as yet unknown) signaling cues expressed by the lung and/or the gut. How NCC discriminate between signals such as Ret/Gdnf in the gut and lung remains to be determined but differing levels of Ret expression by these cell types may be important, as has previously been shown for vagal and sacral NCC that differ in their ability to colonize the gastrointestinal tract [38].
Another possible mechanism for facilitating migration of NCC from the gut into the lung is via the vascular system, which has been suggested to act as a substrate for the directed migration of NCC in the gut [39]. In support of this idea, the vascular system begins to form within the developing lung buds concomitant with the migration of vagal NCC into the lung [28]. However, our recent studies in chick embryos do not support this idea, as it was found that in the lung the innervation and the vascular system initially develop on opposite sides of the lung buds, clearly establishing two distinct early spatiotemporal patterns [28] (Fig. 4.3a–c). Interestingly though, the nerve and vascular networks are found in very close proximity at later stages of development , suggesting that neurovascular units within the lungs are functionally important (Fig. 4.3d) [28].
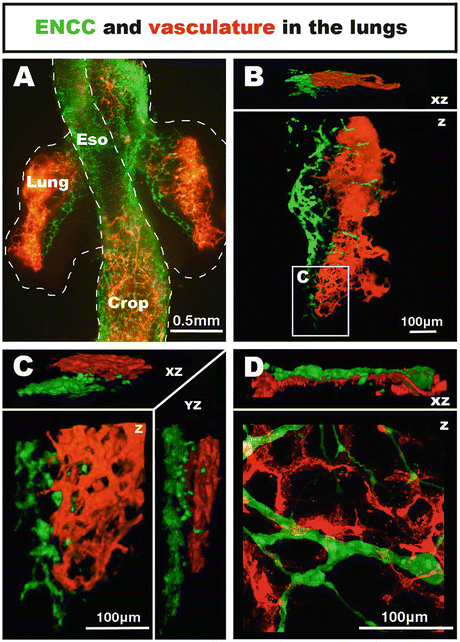
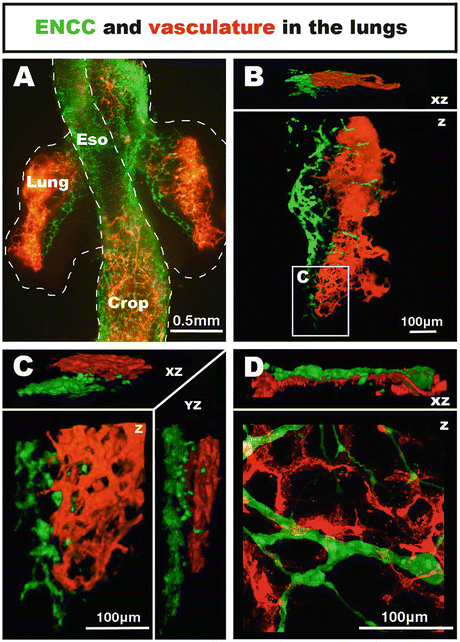
Fig. 4.3
Co-development of neural crest-derived innervation and associated vasculature in the embryonic chicken lungs. (a–c) Wholemount preparation of the lungs at E5.5. NCC are located within the mediodorsal region of the lungs, whereas the vascular system is located on the lateral side. (d) High magnification confocal image of the lungs at E11.5 (HH37–38). NCC form a thin ganglionated network intertwined with the vascular system. From [28]
Throughout their early development , lung NCC are closely associated with the developing vagus nerves (see Fig. 4.2). Tollet et al. using a p75NTR antibody to label neural crest-derived cells in mouse embryos, revealed NCC in the vagus nerve and in processes extending from the vagus to the lung [15]. This suggests that NCC may also migrate along these paired extrinsic nerves to gain access to the lung. Indeed, following preestablished nerve fibers has already been recognized as a migratory behavior for another population of NCC, the sacral NCC, which contributes to the hindgut ENS. Studies on chick [40, 41] and mouse [42] have shown that the sacral NCC, which are known to share molecular signatures with vagal NCC [38], follow already established nerve projections from the extrinsic nerve of Remak in the chick, or pelvic plexus in the mouse, as a route for gaining entry to the hindgut. Moreover, this nerve-associated migratory behavior seems to be more common than previously recognized. In particular, a recent study from the Brunet laboratory showed that migrating cranial NCC can only form parasympathetic ganglia after being guided to the site of ganglion formation by nerves laying down fibers in “preganglionic” territories [43]. Interestingly, it can also be noted that an organ like the liver, which is only innervated by extrinsic nerve fibers after the initial wave of NCC migration along the gut has passed, does not contain any intrinsic ganglia [44]. Together, these studies suggest that preestablished neural projections offer an additional pathway for migrating NCC to colonize the lungs and that an absence of projecting nerve fibers could even prevent NCC entering an organ, such as the liver, during early development. However, detailed analysis of the interrelationships between intrinsic and extrinsic innervation is hampered by the fact that there are no known developmental models with a selective deficiency in intrinsic lung neurons, and by a lack of molecular markers that distinguish intrinsic from extrinsic neurons.
Development of Extrinsic Lung Innervation
As discussed above, extrinsic pulmonary innervation begins to develop during embryonic stages concomitant with intrinsic lung innervation. The extrinsic innervation of the lung, which will allow direct bronchopulmonary communication with the central nervous system (CNS), arises from the vagal and sympathetic nerve trunks of the autonomic nervous system. A proportion of extrinsic lung innervation, the vagus nerve fibers from the vagal nodose ganglia, is derived from the nodose epibranchial placode [45]. Other sensory innervation, including that from the dorsal root ganglia, vagal jugular ganglia, and cervical thoracic ganglia, is neural crest-derived [46]. The vagal fibers that innervate the mouse lungs have been shown to contain a mix of placode-derived and neural crest-derived fibers [47].
Parasympathetic innervation from the brainstem, nodose ganglia, and jugular ganglia enters the lung via the vagus nerve, while sympathetic pulmonary innervation comes from the dorsal root ganglia and cervical thoracic sympathetic ganglia [48]. In contrast to intrinsic lung neurons, whose numbers have been reported to be reduced in Ret mutant mice [20], ASM innervation in the distal lung remains unaffected in these mutants, suggesting that extrinsic lung innervation may require a different neurogenic signal [21, 49]. Studies of E13.5 mouse embryos have shown that extrinsic axons extend into the distal lung and are present in the vicinity of developing ASM [15]. This association between ASM and growing axons is maintained during later embryonic stages and elaborates into a complex neural network closely associated with the smooth muscle [15]. This temporal and spatial interrelationship between axon outgrowth and ASM expression led to the investigation of potential ASM-derived neurotrophic factor(s) coordinating these two aspects of lung development [49, 50]. Xingbin’s group showed that, as Shh signaling induces the specification of ASM, it also represses an inhibitory microRNA (miR-206), which leads to the activation of Brain-derived-neurotrophic-factor (BDNF) expression in the smooth muscle. BDNF is then used as an ASM-derived neurotrophic signal by the extrinsic neurons to extend their axons into the distal lung [49]. In accordance with this result, a study using TrkB (the receptor for BDNF) null mice, showed that the lungs of these mutants had thinner bronchial epithelium , a larger airway luminal diameter, and larger alveolar spaces than their wild-type siblings. This suggests that BDNF/TrkB is important for cell proliferation and/or migration in the lung and ultimately for adequate development of epithelium and ASM [50, 51].
Again, as for the NCC that migrate from the gut into the lung , the guidance cues that direct axons into and within the lungs are elusive, although parallels can be made with signals guiding extrinsic axons innervating the gut. In this organ vagal sensory axons have been reported to respond to chemoattractive netrin signaling cues mediated through the receptor Deleted in Colon Cancer (DCC) as shown in in vitro experiments. Vagal axons in the gut terminate their response to netrin in the presence of laminin, expressed in the extracellular matrix of the gut, suggesting a possible mechanism for vagus nerve terminal positioning [52].
Developmental Interactions Between the Airway Innervation and Its Cellular Targets
As already mentioned, ASM is one of the main targets for lung innervation. ASM has been suggested to have a potential role in the antenatal development and growth of the lung [53]. The idea is that the peristaltic contraction of ASM moves fluid through the developing lung [54] and that the pressure of the fluid against the lung tissue may provide a mechanical stimulus that further promotes lung growth [55]. Evidence for this comes from studies showing that increasing intraluminal pressure within the lung stimulates epithelial branching in culture and may increase lung growth in vivo [56]. In the absence of positive internal pressure, lung growth is decreased [29]. In further support of the possible link between embryonic ASM peristalsis and lung growth, ASM dysfunction has been linked to hypoplastic lung growth [57]. Also, ASM dysfunction occurs in, and may cause, lung hypoplasia in a congenital diaphragmatic hernia (CDH) rat model, where lung hypoplasia precedes CDH [58]. This peristaltic movement of ASM appears to be initiated and maintained independently of neuronal input [59]. Intrinsic calcium channel activity is important in maintaining ASM contractility prenatally [60] and tone postnatally [59], but the role of lung innervation in driving ASM peristalsis has not been investigated. ASM contraction is mediated by intrinsic calcium currents and appears to be modulated by neuronal input [61].
Other, (trophic) interaction(s) between the developing lung innervation and cell types such as the ASM have not been studied extensively during development , but an interesting parallel can again be made with the gastrointestinal tract. In particular, the innervation of the stomach develops in a similar manner to that of the lungs with a contribution from vagal NCC that give rise to intrinsic ganglia, as well as extrinsic innervation from the vagus nerves [62]. Recent work by Faure et al. [63] highlighted the importance of enteric NCC in regulating smooth muscle patterning and differentiation in the stomach. Specifically, results suggested that a crucial number of enteric NCC is required for maintenance of smooth muscle identity and differentiation. These authors demonstrated that experimental reduction of the numbers of enteric NCC induced sustained activation of the BMP and Notch pathways in the stomach mesenchyme, which impaired smooth muscle development [63]. Considering the importance of the Notch pathway in multiple aspects of lung development (as reviewed in [64]), this type of muscle developmental regulation by a neural component could also be important for ASM development in the lungs, but as yet this remains only a tantalizing hypothesis.
Another important target of lung innervation is the neuroepithelial bodies (NEB). Pulmonary neuroendocrine cells (PNECs), also called neuroepithelial endocrine cells, are hypoxia-sensitive chemoreceptors found in the lung airway epithelium adjacent to the lumen of the airway (reviewed in [65–68]). They express the calcitonin neurotransmitter marker CGRP [69] and unlike intrinsic pulmonary neurons are not derived from the neural crest, instead arising from the endoderm that forms the airway epithelium [70]. Some, but not all, PNECs aggregate with other PNECs to form specialized structures innervated by multiple extrinsic nerves, and are termed NEBs [66]. During lung epithelium development , different assemblies of Notch receptors coordinate the number and distribution of NEBs in the conducting airways [71]. NEBs are also sites of airway epithelial stem cell reservoirs [72]. NEBs are innervated by vagal afferents, which transmit information to the CNS, as well as dorsal root ganglion afferents that may modulate intrinsic neuron activity via axon reflexes. Intrinsic neurons are found in close proximity with NEBs and may influence their responses by releasing nitric oxide (NO) [68]. In the mature lungs, the role of NEBs is to respond to intraluminal stimuli, including pO2 and pCO2, pH of the airway surface fluid, and environmental stimuli such as nicotine [68]. An increase in the density of NEBs has been reported in lungs from cases of sudden infant death syndrome (SIDS) [73, 74]. One of the possible consequences of this reported increased density of NEBs is increased sensitivity to hypoxia. The control of proliferation of NEBs and PNECs is thus potentially important in early respiratory function and, as we will see in the following paragraph, is likely influenced by the co-development of the neuronal network.
Only a few studies have investigated potential interactions between the developing extrinsic innervation with other cells type during lung development . Most noteworthy is a recent paper, using the 2-photon laser ablation technique targeting the local parasympathetic ganglia in organ culture, which demonstrated that elimination of these ganglia abolishes lung branching and diminishes endothelial and epithelial cell proliferation [75]. Using drosophila, Bower et al. were also able to demonstrate that this neural control of airway morphogenesis is conserved between invertebrates and mammals, highlighting the importance of this developmental interaction. Prior to this finding, other studies have also suggested that parasympathetic nerves are a vital component of the progenitor cell niche during development , maintaining a pool of progenitors for organogenesis [76, 77]. Using keratin-5 as a marker for epithelial progenitor cells , Knox et al. demonstrated that the removal of the parasympathetic ganglion decreased the number and morphogenesis of progenitor cells in mouse embryonic submandibular gland culture. Moreover, the study also showed that this regulation of epithelial development is controlled via the acetylcholine-signaling pathway [76]. This is significant as embryonic submandibular glands share many of the features of lung development, including branching morphogenesis, NCC colonization and development of an extensive parasympathetic and sympathetic extrinsic innervation [78, 79].
Insight from Developmental Defects Affecting Airway Innervation
As mentioned above, the biggest hurdles preventing a better understanding of the role of lung innervation during development are (1) that there are no known developmental (mouse) models with a selective deficiency in intrinsic lung neurons and/or extrinsic innervation, and (2) that there is a lack of molecular markers that distinguish intrinsic from extrinsic neurons. One possible route for exploring these processes is analyzing human conditions or congenital abnormalities that impair lung function and where there is at least some understanding of the genes and signaling pathways underlying the defects. Some of these conditions are briefly discussed and listed below.
Central Congenital Hypoventilation Syndrome and Phox2b
Central congenital hypoventilation syndrome (CCHS) is a disorder that results in an absence of adequate autonomic control of respiration with decreased sensitivity to hypoxia and hypercapnia. In CCHS respiration slows dramatically during sleep and does not increase in response to hypoxia, causing suffocation. CCHS is primarily considered a disorder of central respiratory pattern generation and can cause death before the age of 2 years (reviewed in [80]). Mutations in PHOX2B are strongly associated with CCHS in humans [80–83] and have a similar effect on respiration in mouse models [84].
Phox2b is a paired homeobox transcription factor expressed by neurons in the central and peripheral nervous system [85]. It is necessary for the development of much of the autonomic nervous system, including neural crest-derived peripheral autonomic innervation and neural crest-derived intrinsic enteric neurons [86, 87]. Phox2b is also important in the development of neurons in the brainstem that regulate ventilation in response to carbon dioxide levels [88]. There also exists a weaker association between CCHS and RET mutations, in which RET mutations increase the risk of intestinal aganglionosis when accompanying PHOX2B mutations [89]. Phox2b has been reported to regulate the downstream expression of Ret and Gdnf [86]. As Ret −/− mice have aganglionosis due to failure of NCC to colonize the entire gut and also a depressed ventilatory response to inhaled CO2 [36], there may be an, as yet unidentified, link between NCC development and breathing control.
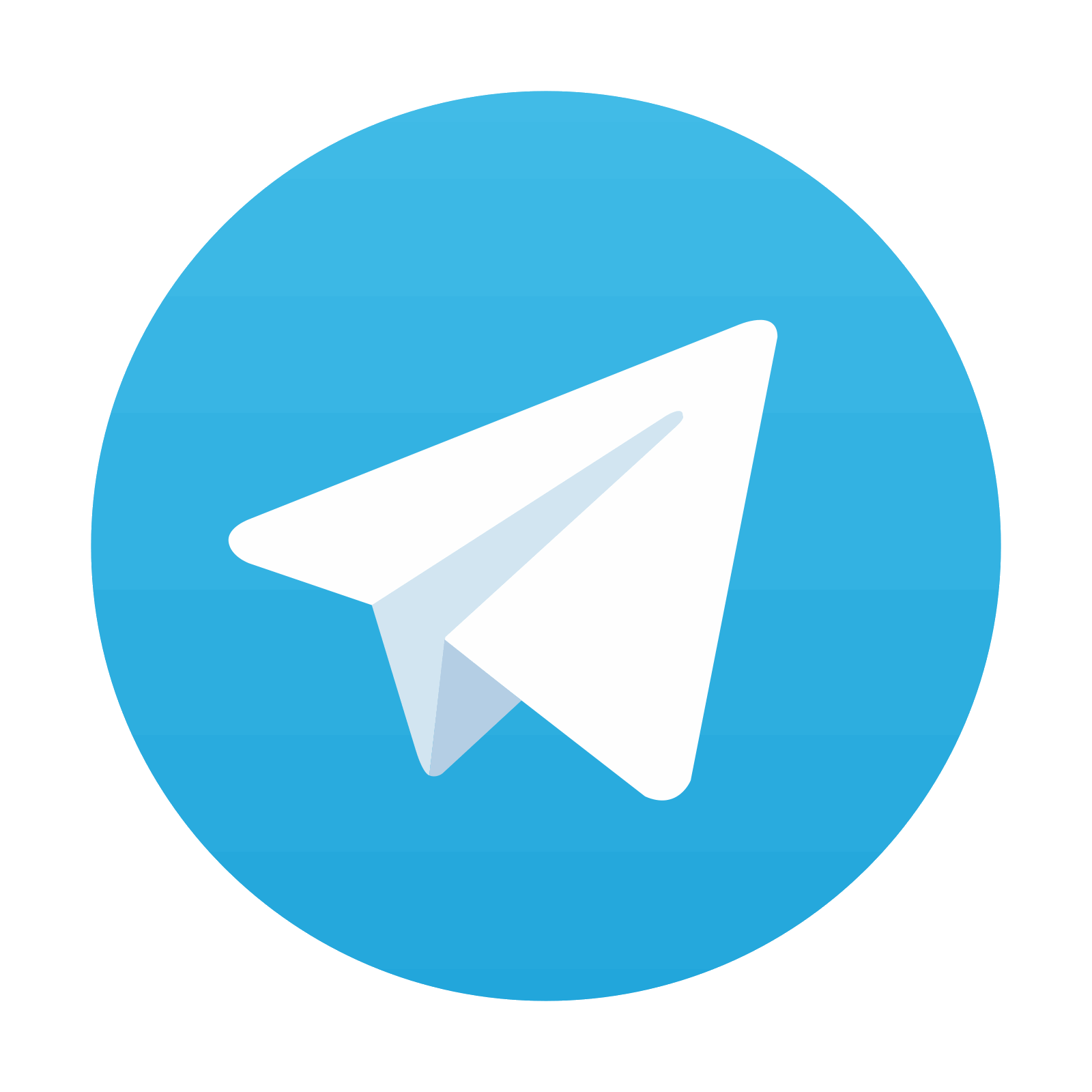
Stay updated, free articles. Join our Telegram channel
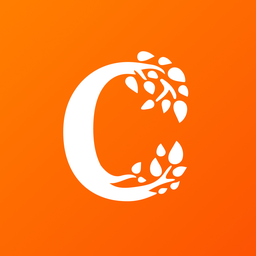
Full access? Get Clinical Tree
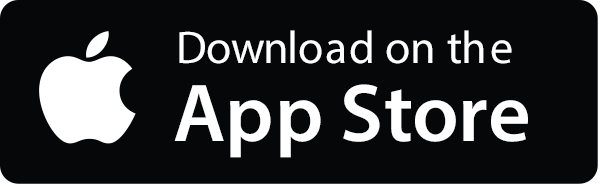
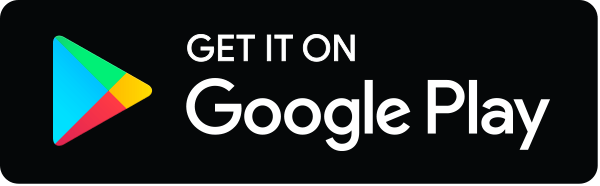