Key Points
- •
Atherogenesis and contributing factors may be conceptualized in steps, including initiation of endothelial activation and inflammation, promotion of foam cell formation, progression of complex plaques, and precipitation of acute events.
- •
Redundant signaling pathways lead to endothelial activation in areas of slow flow (especially with flow reversal) and a quiescent endothelial phenotype in areas of higher, unidirectional flow, providing an explanation for predictable locations of atherosclerosis-prone sites.
- •
Dyslipidemia can lead to endothelial activation through several redundant pathways including LOX-1, TLR4, RAGE, and possibly inadequate protection from HDL.
- •
Numerous cytokines and chemokines binding to their cognate receptors (many with redundant or overlapping roles) direct accumulation of subendothelial macrophages and other leukocytes.
- •
Multiple lipoprotein modifications, including nonoxidative, are likely to contribute to foam cell formation in atherosclerotic lesions.
- •
Knockout studies in mice usually have modest effects on atherosclerosis, perhaps because of redundancy in the pathways.
- •
The difficulty of demonstrating human genetic associations and their modest effects documented to date (other than the few genes that strongly affect major risk factors) may be explained, in part, by the redundant nature of pathways involved in atherogenesis.
During the past 10 to 15 years, there has been an explosion of knowledge regarding the molecular basis of many diseases, including atherosclerosis. Indeed, the various aspects of atherogenesis illustrate most of the major themes of contemporary molecular biology and cell signaling. Whereas some reluctance to delve into the complexities of these pathways is natural, it is reassuring that many of these signaling pathways and their oddly named members are becoming canonical. Indeed, knowledge of at least some of this newer information will become ever more necessary. This chapter emphasizes the molecular biology and genetics of atherosclerosis, with little discussion of the arguably equally important genetic determinants of the major risk factors.
Perhaps one of the major insights to be gleaned from consideration of the various pathways involved in atherogenesis is their sheer number and complexity. Advances in the science of intracellular signaling and a growing appreciation for the number of cytokines, chemokines, and receptors involved in cellular communication during atherogenesis led this reviewer to recognize one remarkable feature of such systems— redundancy . Redundancy is seen as a means to ensure the operation of critically important pathways even when one or more elements may be dysfunctional. Thus, a similar element may take over the function of another element or provide a slightly different but overlapping utility. Redundancy is particularly evident in pathways important for survival, on both a cellular and an organismal level. In this regard, atherosclerosis shares numerous pathways involved in defenses against pathogens, inflammation, and cell survival. As it turns out, these pathways are characterized by redundancy (see Table 8-1 ).
Gene | Effect † | Gene Function |
---|---|---|
PKCβ1/2 | ↓↓ | A proinflammatory conventional PKC |
Egr-1 | ↓↓ | Proinflammatory transcription factor activated by JNK |
PECAM-1 | ↓ to ↓↓ | Flow sensor, involved in intercellular junction |
Fibronectin, EIIIA | ↓↓ | Extracellular matrix component, proinflammatory |
Cx37 (BMT) | ↑ | Regulates macrophage adhesion to endothelium |
Cx43 | ↓↓ | Promotes leukocyte accumulation |
eNOS | ↑ | Synthesis of NO |
p47 phox | ↓↓ | Critical component of NADPH oxidase |
Renin | ↓↓ | Produces angiotensin I from angiotensinogen |
AT1R | ↓↓ | Angiotensin receptor 1 (can activate NADPH oxidase) |
HO-1 | ↑↑ | Important cellular enzymatic antioxidant |
MnSOD | ↑↑ | Important cellular enzymatic antioxidant |
PRDX1 | ↑ | Important cellular enzymatic antioxidant |
LIAS | ↑ | Synthesis of lipoic acid, a mitochondrial antioxidant |
NEMO | ↓ | Component of IKK that phosphorylates IκB |
IκB (DN) | ↓↓ | Sequesters NF-κB in cytoplasm until phosphorylated |
TLR2 | ↓ | A toll-like receptor—normally recognizes PAMPs |
TLR4 | ↓↓ | A toll-like receptor, binds modified lipoproteins |
MyD88 | ↓↓ | Adaptor protein critical for TLR signaling |
LOX-1, OLR1 | ↓↓ | Activation of endothelial cells by modified lipoproteins |
RAGE | ↓↓ | Activation of endothelial cells by modified lipoproteins |
PARP-1 | ↓↓ | DNA repair and stress enzyme–activated DNA damage |
ROCK1 | ↓↓ | Transmits contraction signals from Rho |
PI3Kγ, p110γ | ↓↓ | Regulates macrophage chemotaxis |
5-LO | ↓↓↓ | Rate-limiting enzyme for leukotriene synthesis |
* In this and the following tables, studies were whole-body knockout of the gene unless otherwise indicated, performed in either apo E–deficient or LDL receptor knockout mice.
† Effects on atherosclerosis (for all tables): ↓, <50% reduction; ↓↓, 50%-80% reduction; ↓↓↓, >80% reduction; ↑, <100% increase; ↑↑, 100% or greater increase.
The question then arises: What will be the expected impact of redundancy on our efforts to find genes related to atherosclerosis risk? Whereas the effects on atherosclerosis of many genes may be clearly evident in highly controlled experiments during the short term, their apparent effects may become lost over time if other mechanisms can substitute for or duplicate their action. If this is the case, redundancy of complex systems may help explain the remarkable difficulty in identifying genes for such complex, common diseases as atherosclerosis and hypertension. Redundancy of the various activation and transduction pathways will be a recurrent theme throughout this chapter.
A General Overview of Atherogenesis
Atherogenesis and its clinical expression may be divided into four major steps. Terminology for this model borrows from the cancer literature and was fairly complete in general outline fully 30 years ago. The steps include initiation of endothelial activation and inflammation; promotion of intimal lipoprotein deposition, retention, modification, and foam cell formation; progression of complex plaques by plaque growth, enlargement of the necrotic core, fibrosis, thrombosis, and remodeling; and precipitation of acute events, primarily through plaque destabilization and acute thrombosis. Note that acute events may be precipitated by factors unrelated to atherogenesis, for example, through mismatch of arterial oxygen supply and myocardial demand (as with heavy exercise in the setting of uncompensated subtotal coronary occlusion and vulnerable myocardium, leading to ischemia and ventricular fibrillation).
In this scheme, factors frequently act at more than one step of atherogenesis, particularly the major risk factors. For example, elevated lipids can contribute to endothelial activation (with or without oxidation), impair nitric oxide (NO) synthesis by endothelium or its availability, lead to foam cell formation (after a variety of possible modifications), increase platelet activation and thrombotic potential, and promote reversible plaque destabilization. Obviously, factors that increase thrombotic potential may also help precipitate an acute event if an occluding thrombus ensues. Factors that initiate inflammation also are likely to lead to an unstable plaque.
During initiation, several key events occur as illustrated in Figure 8-1 . In areas predisposed by hemodynamic factors, atherogenic lipoproteins, including low-density lipoproteins (LDL) and smaller very low-density lipoproteins (VLDL) as well as other remnants of triglyceride-rich lipoproteins (TGRL), infiltrate the intima and are modified by several potential mechanisms. The modified lipoproteins appear to release inflammatory signals to endothelial cells, causing them to become activated. Activated endothelial cells elaborate various chemoattractant and adhesion molecules, such as monocyte chemoattractant protein 1 (MCP-1) and vascular cell adhesion molecule 1 (VCAM-1) recognized by cognate receptors on passing monocytes (and, to a lesser extent, other white cells and platelets) that lead to a sequence of rolling, firm adhesion with spreading and diapedesis or transmigration into the subendothelial layer of the intima. Slow or disrupted flow is a major determinant of endothelium activation.

Once in the intima, monocytes transform into activated macrophages capable of ingesting the modified lipoproteins and further amplifying the inflammatory response. Concomitantly, inflammatory cytokines, such as platelet-derived growth factor (PDGF) from adherent platelets and other cells, summon smooth muscle cells to move into the intima, where they change from a contractile to a synthetic phenotype capable of ingesting modified lipoproteins, synthesizing and secreting collagen, and producing various cytokines. Note that platelets can adhere directly to activated endothelial cells, much as white cells do ; desquamation of the endothelium is not a prerequisite. T cells and mast cells also participate importantly in immune signaling and amplification. Thus, during initiation, the intima becomes populated with inflammatory cells poised to do battle with the modified lipoproteins, which are seen as foreign invaders in unauthorized territory.
During the promotion phase ( Fig. 8-2 ), insudation of lipoproteins and their modification continue in proportion to their plasma levels, endothelial permeability, and transarterial pressure gradient that drives fluid convection. Unchecked uptake of remnants or modified lipoproteins by several scavenger receptors on macrophages and smooth muscle cells leads to formation of foam cells, the hallmark of the growing atherosclerotic lesion. Macrophage foam cells remain capable of relatively rapid egress from the lesion if conditions are favorable (such as a marked reduction in serum lipoprotein concentration) but seem to be retained when lipid levels are high. Thus, if the balance between lipoprotein entry, foam cell formation, reverse cholesterol transport, and foam cell egress favors cholesterol accumulation, the lesion grows. If excess free cholesterol accumulates within foam cells (particularly with cholesterol monohydrate crystal formation), both apoptosis and necrosis can occur. This marks the beginning of the formation of the necrotic core as illustrated in Figure 8-2 .

As the lipid-rich plaque progresses ( Fig. 8-3 ), accumulating macrophages secrete a host of cytokines and matrix metalloproteinases (MMPs). All migrating cells secrete MMPs to facilitate their diapedesis through extracellular matrix. Thus, highly cellular plaques would be expected to be more friable. MMPs also undermine the stability of the overlying fibrous cap and contribute to plaque rupture with exposure of the blood to the thrombogenic underlying matrix. In addition, interferon-γ (IFN-γ), secreted by activated T cells, acts to strongly inhibit collagen formation by smooth muscle cells, further weakening the plaque and fibrous cap. The result can be catastrophic thrombosis and downstream tissue infarction; but more often, there is limited mural thrombosis with subsequent organization leading to saltatory growth of lesions. Other precipitating changes in the plaque include erosions and, importantly, eruption through the endothelium of underlying cholesterol crystals. Before such episodes of thrombosis, there may be little if any encroachment of the plaque into the arterial lumen because of outward remodeling of the arterial wall to accommodate the growing plaque. Hemodynamic factors and other risk factors seem to influence remodeling and the percentage of the plaque filled with fibrous tissue versus lipid. Calcification appears to be associated with healing or more fibrous plaques, although the overall coronary calcium score remains a powerful predictor of overall atherosclerosis and subsequent risk for coronary events.

When the intimal thickness increases beyond just 0.5 mm, hypoxia induces ingrowth of vasa vasorum. In recent years, there has been growing appreciation for the potentially major destabilizing effects of these leaky, friable vessels. Vasa vasorum invading lipid-rich plaques remain highly permeable because of constant exposure to inflammatory factors, possibly in a large measure from mast cells. Rather than sudden hemorrhage, a constant leak of red blood cells leads to progressively larger amounts of red cell markers found in unstable plaques. Thus, red cell membranes may greatly exacerbate the accumulation of free cholesterol and promote formation of toxic cholesterol crystals. Furthermore, the red cells are a source of strongly pro-oxidant heme iron, which would favor inflammation and lesion progression generally. Such a scenario may favor progression of lesions for some time even after plasma lipoprotein levels are reduced.
Initiation of Atherosclerosis
Disturbed Flow and the Atherosclerosis-Prone Endothelial Phenotype
In recent years, endothelial response to flow has been the subject of intense investigation. Sites of atherosclerosis predilection occur in areas of low shear or eddy currents ( Fig. 8-4 ) characterized by slow, oscillating (back-and-forth) flow. Such a pattern is referred to as disturbed flow even though the pattern is stable over time in specific areas of the arterial tree. This observation has been confirmed in many observational and experimental studies and is supported by extensive mathematical modeling. Progression of plaque is predictable in individual human subjects at such locations in the arterial tree. Turbulence is virtually never a feature of flow in these sites. Experimentally, atherosclerotic lesions accumulate exclusively in areas of low shear just beyond stenoses created by carotid casts in hyperlipidemic mice. The unique hemodynamics of the coronary circulation, with near cessation of flow or flow reversal during systole, together with the high pressures generated at the aortic root may explain the marked predilection of coronary arteries to atherosclerosis. Interestingly, rabbit coronary artery endothelial cells expressed only one fifth the endothelial nitric oxide synthase (eNOS) and greater endothelin-1 (ET-1) compared with aortic endothelial cells. As heart rate increases, relatively more time is spent in systole, when flow is essentially nil. This may help explain the fourfold increase in coronary artery disease (CAD) risk as resting heart rate increased from below 60 to above 100 beats per minute.

Multiple, redundant transduction mechanisms endow endothelial cells with exquisite sensitivity to abrupt changes in shear stress. These mechanisms include ion channels, G protein–coupled receptors (GPCRs), the lipid bilayer itself, and the endothelial glycocalyx, with stress transmitted throughout the cell by microfibers and sensed by integrins and at cell junctions. In general, more rapid flow induces remodeling, which results in larger vessels. Conversely, slow flow results in diminution in the size of the vessel. In this way, flow becomes a fundamental stimulus for blood vessel development during embryogenesis. In addition, flow strongly affects numerous endothelial responses, including endothelial cell migration, mitosis, apoptosis, endothelial layer permeability, inflammation (including white cell adhesion), NO release, and thrombosis.
The pattern of flow is critical in determining the endothelial phenotype. Direct measurement of flow patterns by ultrasound and magnetic resonance imaging techniques in atherosclerosis-resistant compared with atherosclerosis-prone areas revealed marked differences as shown in Figure 8-5 . Endothelial cells exposed to the atherosclerosis-prone flow pattern, characterized by slow flow that reversed direction slightly during the course of a cardiac cycle (referred to as oscillatory or disturbed flow), developed a proatherosclerotic phenotype, whereas cells exposed to higher flow rates (higher shear stress or laminar flow) that remained unidirectional although pulsatile had an antiatherosclerotic phenotype. The time course of phenotypic changes and some of the molecular signaling events in the endothelial cells exposed to the respective flow patterns are also shown in Figure 8-5 . Platelets and white cells are also much more likely to attach to areas of slow flow, either to endothelium or even when measured for attachment to artificial surfaces such as one coated with E-selectin.

Of great importance is the relatively recent recognition that proinflammatory endothelial responses occur only transiently with onset of flow, after a sudden stepped increase in flow, or with directional change in flow. However, after several hours of continued unidirectional, laminar high shear stress or pulsatile flow (suggesting undulation in the rate of flow but always unidirectional and faster than disturbed, oscillatory flow), these proinflammatory responses are suppressed to below initial (no flow) conditions (see Fig. 8-5 ). After prolonged exposure (10 to 12 hours) to unidirectional flow, the cells thus display an anti-inflammatory, antioxidant, antiproliferative phenotype, remaining in a relatively quiescent state but active in production of protective NO and prostacyclin (PGI 2 ). In contrast, when endothelial cells are exposed to slow oscillatory flow, where direction of flow actually reverses however slightly during the cycle, they never suppress their proinflammatory responses, do not align with the flow, have disorganized cytoskeletons, and develop other features characteristic of lesion-prone areas including increased apoptosis, frequent mitoses, greater permeability (particularly at sites of mitosis), shorter glycocalyx, elaboration of cell adhesion molecules, secretion of MCP-1 and endothelin, decreased bioavailability of NO, and increased production of superoxide by NADPH oxidase and heme oxidase. In addition, these endothelial cells increase production of subendothelial matrix components, such as fibronectin, which results in enhanced inflammatory responses and a thickening of the basement membrane that may be seen as an increase of intima-medial thickness on ultrasound. The classic inflammatory markers nuclear factor κB (NF-κB) and protein kinase Cß (PKCß) as well as other markers of inflammation have been used to track the increased inflammatory signal seen with early exposure to rapid flow or prolonged exposure to slow flow as well as its suppression with longer term exposure to high shear.
Redundant Mechanotransduction and Cell Signaling Mechanisms
A number of generally proinflammatory signaling pathways are activated immediately or soon after abrupt onset of flow ( Fig. 8-6 ). If disrupted flow continues (either low shear stress or back-and-forth flow), the proinflammatory, proatherogenic state described earlier becomes more fully established. A number of genes in these pathways have been subject to knockout or overexpression in atherosclerosis-prone mice. Although a thorough review of each of the affected signaling pathways cannot be undertaken here, an attempt is made to orient the reader to the location and general function of the specific signaling pathway in the endothelial cell to appreciate the general stage of atherogenesis in which a particular gene may function. Note that whereas a number of the following pathways may be considered canonical and dealt with in general reviews of signaling or in textbooks, they may subserve rather different purposes in specific tissues. Emphasis in the following paragraphs is on such signaling in endothelial cells and in the context of disturbed flow.

The endothelial glycocalyx is important in mechanotransduction, particularly in mediating responses seen in the first few minutes after flow change. The glycocalyx is also involved in inflammatory cell adhesion, thrombosis, migration, and endothelial permeability. The endothelial glycocalyx can be thicker (up to 500 nm or more) than the endothelial cell itself (300 to 400 nm). The glycocalyx is composed primarily of the proteoglycan syndecan 1 (which consists of a core protein and three or more glycosaminoglycan chains attached). Syndecan 1 is arranged in a highly structured hexagonal lattice spread over the luminal surface of the endothelial cell with interconnections to the actin cortical web, part of the cytoskeleton. The stiffness of the proteoglycans and their ability to transmit torque to the endothelial cytoskeleton make for an “exquisitely designed transducer of fluid shearing stresses.” Treatment of endothelial cells with heparinase selectively removes heparan sulfate but not the syndecan 1 core protein and markedly disrupts the glycocalyx layer, reducing many but not all flow-induced responses in endothelial cells, such as NO release in response to shear stress.
In the following paragraphs, several pathways of endothelial mechanotransduction are reviewed roughly in the order in which they are activated by onset of shear stress. They include (1) PKC activation through calcium channels and a GPCR; (2) bone morphogenic protein 4 (BMP4) activation with subsequent generation of reactive oxygen species (ROS) and activation of NF-κB; (3) platelet and endothelial cell adhesion molecule 1 (PECAM-1) and integrin activation; (4) activation of MAPK pathways, particularly JNK; and (5) activation of heat shock proteins.
Within a few seconds after onset of blood flow, there is an influx of calcium (e.g., through the polycystin-2 channel), potassium, and chloride through respective ion channels and activation of GPCRs (such as the bradykinin B2 receptor, which is activated without binding of agonists). The resultant G protein signaling activates phospholipase C with production of diacylglycerol and subsequent activation of PKCβ. In addition, there is a sudden initial burst of NO production that is calcium, calmodulin, PKC, and G protein dependent. There may also be a mechanical effect from glypican and CD44 to assist in caveolin-1 phosphorylation and removal of its suppressive effect on eNOS. This stimulation of NO production is generally considered anti-inflammatory and antiatherosclerotic. However, PKC signaling can activate proinflammatory signaling through several MAPK pathways, and knockout of PKCβ resulted in marked reduction in atherosclerosis.
BMP4 activation with subsequent activation of NADPH oxidase and heme oxygenase 1 activity together with accumulation of superoxide and other ROS can lead to NF-κB activation. NADPH oxidase may also be upregulated directly in response to onset of shear stress.
Abrupt onset of flow results in torsional forces transmitted by the glycocalyx to the actin cortical web. This force is thought to be transmitted through actin stress fibers to the dense peripheral actin band and to syndecan 4 and integrins that anchor the endothelial cell to the extracellular matrix. Tractional forces may also be transmitted to lamins on the nuclear envelope. The dense peripheral actin band surrounds the endothelial cell much like a rubber bumper encircles a bumper car. It has been proposed that the tractional forces on this band disrupt the weak links between vascular endothelial cadherin (VE-cadherin) proteins of adjoining cells, resulting in the transmission of a signal that is dependent on PECAM-1, VE-cadherin, and vascular endothelial growth factor receptor 2 (VEGFR-2, also called Flk-1). In this process, PECAM-1 appears to act as the true mechanical transducer that directly activates a Src family kinase and also results in release of bound Gαqr. VE-cadherin functions as an adapter protein allowing Src to activate VEGFR-2, which then activates phosphatidylinositol 3-kinase (PI3K), which in turn activates integrins as indicated in Figure 8-6 . The activated integrins then form new bonds to the extracellular matrix, which triggers activation of Rho, Rac, and Cdc42. In endothelial cells, these work in a coordinated fashion to modify actin polymerization and to mediate cell alignment to flow and other responses. Early changes lead to contraction and increased intercellular permeability. The adapter protein Shc is phosphorylated in response to integrin activation, enabling signaling through other adapters, Grb and Sos, to Ras and Raf with subsequent signaling to the MAPK pathway. Integrin binding and activation also lead to phosphorylation of focal adhesion kinase (FAK), which in turn binds and activates Src, followed by phosphorylation of paxillin and p130 cas , which then activate Ras. Alternatively, FAK may first bind Shc with subsequent activation of Src; a third pathway results in activation of Rho. The kinase Src may also directly transmit signals to the MAPK pathway.
Yet another result of integrin activation is activation of p21-activated kinase (PAK), which may be one of the most important activators of the MAPK pathway resulting from shear stress. This activation appears to depend on PI3K signaling from the PECAM-1, VE-cadherin, VEGFR-2 complex. Not only does PAK activation lead to activated JNK (a terminal MAPK), but it may also directly affect various cell junctional adhesion molecules, resulting in increased endothelial permeability. Importantly, the activation of PAK appears to depend on integrin binding to fibronectin in the extracellular matrix. The protein ZO-1, associated with tight junctions, is also involved in the increase in endothelial permeability after onset of flow or prolonged oscillatory slow flow. Connexin43 (Cx43), unlike other connexins, relocates to cell junctions in response to onset of shear stress and is increased in atherosclerosis-prone areas of the endothelium.
Importantly, there is greater inflammatory response to onset of shear stress when integrins are bound to fibronectin or fibrinogen (characteristically found in extracellular matrix underlying previously injured endothelium) compared with components of the normal extracellular matrix unexposed to prior injury. This difference in inflammatory response seems to be mediated by the binding specificity of different integrins, but activation of Akt also seems to play a role. The alteration of the extracellular matrix, with increase in fibronectin, occurs early in atherosclerosis-prone areas as part of the cellular response to disrupted flow, whereas deposition of fibrinogen may occur later.
As noted before, several of the signals activated on abrupt onset of flow converge on the MAPK/ERK (mitogen-activated protein kinase/extracellular signal-regulated kinase) or simply MAPK pathway, which results, ultimately, in activation of a variety of transcription factors, including Egr-1 (early growth factor 1) and AP-1 (activator protein 1, which contains c-fos and c-Jun). NF-κB may also be activated by crosstalk with MAP kinases.
The canonical MAPK pathway represents a generic designation for a series of three kinases, often held in juxtaposition by a scaffolding protein (with other regulatory proteins sometimes attached, such as IMP or “impedes mitogenic signal propagation”). MAPK designates the terminal kinase. It is activated by a MAPK kinase (MAPKK), also called a MAPK/ERK kinase (MEK). This intermediate kinase is phosphorylated by an upstream MAPKK kinase (MAPKKK or MAP3K) also known as a MEK kinase (MEKK). The MAPK pathway or complex is activated by upstream kinases often in response to tyrosine receptor kinases (classically worked out for EGFR, the endothelial growth factor receptor) or kinases interacting with integrins or other flow sensors. In the canonical EGFR pathway, after binding endothelial growth factor, the receptor self-phosphorylates its cytoplasmic domains, allowing binding of one or more adaptor proteins (e.g., Grb2, which binds directly to the receptor or through another adaptor, Shc). Grb2 interacts with the guanine nucleotide exchange factor Sos (son of sevenless), which then activates membrane-bound Ras by promoting exchange of GDP for GTP. Once bound by GTP, Ras is active. In this example (for EGFR), active Ras leads to activation of the protein tyrosine kinase RAF1. RAF1 is actually a MAP3K, the first of a series of three kinases leading to activation of the MAPK ERK. A variety of potential kinases may serve as the initial kinase, MAP3K or MEKK (such as RAF1), as well as multiple potential intermediate kinases and terminal kinases. Mammalian terminal kinases include ERK(1-7), JNK(1-3), and p38(α, β, δ, and γ). Traditionally, ERKs are the terminal kinases for mitogens; p38 and JNK result from stress or cytokine activation. Once activated, terminal kinases can phosphorylate membrane proteins, transcriptional factors in the nucleus, proteins associated with the actin cytoskeleton, various additional signaling molecules, and MAP kinase–activated kinases (MK), which in turn have a series of substrates (such as transcription factors, cell survival, and other signaling proteins). Some examples are given in Figure 8-7 . Their control and signal termination are also complex. In endothelial cells, terminal kinases in MAPK/ERK signaling pathways can phosphorylate eNOS and may have diverse other effects on cell function. Whereas p38 and JNK are generally proinflammatory, ERK5 appears to have multiple anti-inflammatory effects.

Another consequence of early-onset shear stress is activation of heat shock proteins (HSPs). These are generally considered protective proteins, and recent evidence suggests that an increase in HSP-90, which can act to stimulate eNOS, is brought about not only by prolonged rapid flow but also by statins as a beneficial, pleiotropic effect. HSPs can act as protein chaperones to promote proper folding (especially HSP-70) and other protective functions. HSP-60 may act to chaperone certain cytosolic proteins into the mitochondria. When cells are not under stress, HSPs are bound to heat shock transcription factor 1 (HSF1) in the cytoplasm. When misfolded proteins are present or the cell is stressed by a number of factors, including ROS, oxidized lipids, or cytokines, the HSP binds to the misfolded protein (or is otherwise used in the cell), thus becoming separated from HSF1. HSF1 then forms a trimer, moves to the nucleus, and stimulates transcription of HSPs. In the case of HSP-60, levels are clearly upregulated in endothelial cells in atherosclerosis-prone areas of slow flow, with increased expression on the luminal membrane. Cell surface HSP-60 may be important in triggering an autoimmune response that may be important in promoting atherosclerosis (see later).
Transition to an Atherosclerosis-Resistant Endothelial Phenotype
After several hours of exposure to laminar flow, the quiescent state described earlier is induced, the actin cytoskeleton organizes itself into a pattern such that the cells align themselves to be elongated in the direction of the flow with tight cellular junctions, and most cells are found in an arrested state of growth (G 0 or G 1 ). Not only are shear-stressed endothelial cells relatively quiescent, but they become frankly resistant to even potent inflammatory cytokines such as tumor necrosis factor-α (TNF-α). The molecular mechanisms that mediate this transition have been the subject of intensive investigation.
Some of the complex intracellular events that appear to underlie the transition from an activated to a quiescent state are depicted in Figure 8-7 . Of great importance is the recent recognition that the organized sequence of protective events is at least in part dependent on an initial burst of ROS produced together with increased NO. At the onset of flow, there is a marked increase in production of superoxide anion (·O 2 − ) by NADPH oxidase, mediated possibly by increased activation of the small GTPase Rac (produced by mechanotransduction mechanisms reviewed earlier) or direct effects transmitted through the cell membrane. At the same time, there is a surge in NO production by eNOS due to calmodulin binding and phosphorylation by PKCβ. Surprisingly, Rac appears to be required for normal expression of eNOS as well. Continued and enhanced production of NO is one of the features of the atheroprotective state. This seems to be mediated by a gradual increase in eNOS mRNA as well as by multiple post-transcription changes in the eNOS enzyme (such as binding to HSP-90, calcineurin, and certain phosphorylations) that decrease the dependence of eNOS on calcium and calmodulin and increase overall activity.
Because ·O 2 − reacts rapidly with NO to form peroxynitrite (ONOO· − ), there is a balance between NO and ·O 2 − production that may be significant for signaling events. Excess or prolonged ·O 2 − clearly has detrimental effects and can promote cellular damage and apoptosis. Nevertheless, more controlled release of ROS may be an important signaling mechanism that is key to subsequent protective adaptations by the cell. Early after onset of laminar flow, or with prolonged slow, oscillatory flow, the balance favors ·O 2 − production with neutralization of vasodilating effects of NO; after several hours of laminar flow, NO is favored through multiple mechanisms. However, some of the vasodilation seen immediately after initiation of flow is mediated by hydrogen peroxide (H 2 O 2 ), which acts as an “endothelial derived hyperpolarizing factor.” In addition, NO can inhibit mitochondrial electron transport chain complex I and IV and thereby at least transiently increase mitochondrial production of superoxide anion. Lipid oxidation products, such as 4-hydroxy-nonenal, potentially produced during the ·O 2 − spike, are taken up actively into mitochondria, where they also promote ·O 2 − production. In the cytosol, ·O 2 − is rapidly converted to H 2 O 2 by copper/zinc superoxide dismutase (Cu/Zn SOD); in the mitochondria, this function is performed by manganese superoxide dismutase (MnSOD). Much of the subsequent “redox signaling” is likely to be mediated by H 2 O 2 , as it is more stable and freely membrane permeable, unlike ·O 2 − or other free radicals. Nevertheless, H 2 O 2 , ·O 2 − , ONOO· − , and hydroxyl radical (·OH) can all contribute to a pro-oxidant environment sensed, in part, through effects on free -SH groups on cysteine residues in various signaling proteins.
Cellular antioxidant defenses depicted in Figure 8-7 include not only the SOD enzymes but catalase, various glutathione peroxidase enzymes, and glutathione- S -transferases (GSTs, which not only convert superoxide to water but reduce a number of other toxic, oxidized molecules by coupling with the GSH to GSSG reaction) as well as glutathione reductase, peroxiredoxins (PRDXs), and thioredoxin (Trx), which regenerate reduced glutathione. Trx also acts as a signaling “gate” by binding (when Trx is in the reduced form) to the MEKK ASK1 (apoptosis signal-regulating kinase 1). Thus, ASK1 is a redox-responsive MEKK. ASK1 appears to be the major mediator of TNF-induced JNK activation in endothelial cells. JNK, as noted before, has major proinflammatory and apoptotic effects. When ASK1 is bound by Trx, it is unresponsive to upstream signals, such as those generated from the binding of TNF-α to its receptor. Trx-bound ASK1 is also targeted for ubiquitination and degradation. Trx is highly responsive to ROS and is released from ASK1 on early exposure to the onset of flow or oxidative stress or during prolonged slow or disturbed flow. In addition, Trx is bound by thioredoxin-interacting protein (TXNIP), which inactivates Trx. Importantly, TXNIP is downregulated by prolonged laminar flow through an unknown pathway. Finally, NO can bind to Trx and increase its binding to and inactivation of ASK1.
A key, critically protective pathway that is stimulated by flow in endothelial cells and the early ROS burst involves the induction of numerous antioxidant genes. Many of these genes (which include most of the antioxidant enzymes depicted in Figure 8-7 ) share an antioxidant responsive element (ARE) in their promoters and are activated by the transcription factor Nrf2 (nuclear factor erythroid 2–like related factor 2). Importantly, Nrf2 is activated by both disturbed and laminar flow, but only laminar flow results in increased expression of the antioxidant genes. This was thought to be mediated in part by the oxidized lipid d15-PGJ 2 generated through cyclooxygenase 2 (COX-2). Nrf2 is bound in cytoplasm by an inhibitor, Keap1 (Kelch-like erythroid-derived cap’n’collar homology–associated protein 1). Keap1 binding targets Nrf2 for proteosomal degradation. Recently, a mechanism was proposed that is dependent on early stimulation of superoxide and hydrogen peroxide by mitochondria, increased early production of NO, and activation of Akt, which all converge to inactivate Keap1 and to release Nrf2 for transport to the nucleus. Effects of oxidized lipids also seem to accelerate the pathway. Direct binding of NO to Keap1 and phosphorylation by Akt appeared particularly important. Critically important for the Nrf2 pathway is the recently discovered effect of KLF2 to prime Nrf2 for greater upregulation of ARE-responsive genes (see later). This interaction, affecting dozens of genes, is one of several major effects promoted by shear stress and KLF2.
The early post-flow, pro-oxidant state promotes signaling through ASK1 and promotes activation of inhibitor of κB (IκB) kinase (IKK). IKK phosphorylates IκB, thereby releasing IκB from NF-κB, targeting IκB for proteosomal degradation, and freeing NF-κB to be transported to the nucleus, where it acts as a transcription factor for numerous proinflammatory genes. As noted before, NF-κB expression is frequently found to be increased in atherosclerosis-prone regions (see Fig. 8-6 ). Nevertheless, NF-κB also acts early to support an increase in eNOS synthesis and has antiapoptotic effects. This yin-yang behavior is modified in the setting of prolonged laminar flow, in which the proinflammatory actions of NF-κB are almost entirely abrogated or “uncoupled” (as seen by a marked suppression of VCAM-1, E-selectin, and IL-8 production in response to TNF-α), leaving the cytoprotective effects of NF-κB (such as an induction of MnSOD and eNOS) intact. Recent insights into the mechanism of this transition suggest activation by Akt (also activated early after the initiation of flow) of a histone acetylator, p300 (generally referred to as p300/CBP; CBP refers to the p300 homologue CREB-binding protein). p300/CBP may also be activated by a short burst of oxidant stress. The activated p300 acts as a coactivator with NF-κB and greatly affects NF-κB activation of genes, especially seen as a marked increase in eNOS transcription. In addition, there is negative feedback exerted by NO at several steps of the NF-κB pathway. Thus, nitrosylation inhibits IKK, stabilizes IκB, induces IκB mRNA, decreases NF-κB transport to the nucleus, and inhibits NF-κB binding to DNA.
Not only does NO provide feedback inhibition to NF-κB, but it also appears to be critical for downregulation of NADPH oxidase subunits after prolonged exposure to laminar flow. Thus, when endothelial cells were subjected to shear forces in a cone-and-plate viscometer, NO and ·O 2 − production were upregulated 6-fold and 2.5-fold, respectively, by 2 hours. There was no change in NADPH oxidase subunit number at this point. It is possible that a higher earlier peak for ·O 2 − was missed. Thereafter, ·O 2 − declined until it was about 50% of the initial level after 24 hours of laminar shear stress while NO production had increased markedly. The investigators found that there was an approximate 50% decrease in the expression of the activity-limiting gp91 phox subunit of NADPH oxidase while eNOS protein expression was increased 3.5-fold. The reduction in gp91 phox expression was shown to be NO dependent, although the specific signal transduction pathway was not demonstrated. Interestingly, endothelial expression of the angiotensin type 1 receptor (AT1R) is also downregulated by shear stress and NO signaling.
Crosstalk between different MAPK pathway kinases appears to be another important mechanism whereby the protective endothelial phenotype is brought on by laminar flow. As depicted in Figure 8-7 , activation of MEK5 by flow inhibits activation of the proapoptotic, inflammatory MAPK JNK. Furthermore, MEK5 activates ERK5, which interferes with signaling downstream of JNK. Perhaps even more important is the induction of KLF2 (Kruppel-like factor 2) by the transcription factor MEF2C (myocyte enhancer factor 2C), which is activated by ERK5. ERK5 also phosphorylates and thereby inactivates the proapoptotic factor Bad.
Numerous studies have pointed to the induction of KLF2 as a critical step in the conversion to and maintenance of an atheroprotective state. KLF2 is expressed almost exclusively in areas of the vasculature protected from atherosclerosis, whereas it is nearly absent in atherosclerosis-prone areas and is clearly upregulated by laminar flow. It is also important in embryonic vascular development. The molecular effects of KLF2 are protean and include: inhibition of activating transcription factor 2 (ATF2), which can be one of the heterodimeric components of AP-1, the key product of the MAPK p38 and a required activating factor for many proinflammatory effects of NF-κB ; induction of inhibitory Smad7, which blocks transmission of proatherosclerotic signals through the transforming growth factor-β (TGF-β) receptor, together with reduction of nuclear c-Jun, a second component of AP-1, thereby further blocking many inflammatory signals ; enhanced transcription of eNOS and the enzyme dimethylarginine dimethylaminohydrolase, which degrades the eNOS inhibitor ADMA (asymmetric dimethylarginine); increased transcription of protective thrombomodulin (TM); inhibition of ET-1 and MCP-1; and other major effects. In one study, KLF2 decreased the expression of the following genes by 80% to 90% after exposure of cells to IL-1β: IL-1α, IL-1β, IL-6, IL-8, IL-15, MCP-1, E-selectin, TNF-α, CXCL10, CXCL11, IFN-γ, COX-2, and CCL5.
An important mechanism to upregulate KLF2 involves activation of p300/CBP-associated factor (PCAF) by a PI3K-dependent but Akt-independent mechanism. Activated PCAF acts together with p300 to acetylate histones and greatly increase transcription of KLF2-regulated genes. Increased PCAF activity is also related to induction of COX-2 independent of KLF2. Thus, PCAF activation appears to be yet another critical mechanism linking early signaling events to the onset of flow. Interestingly, statin drugs are potent inducers of KLF2, possibly by way of Akt activation, a finding that lends credibility to the potential importance of so-called pleiotropic effects of these drugs.
MAPK phosphatase 1 (MKP-1) is another gene found to be expressed exclusively in arterial regions protected by high laminar flow. As MKP-1 deactivates p38 and JNK by dephosphorylating these terminal MAP kinases, it also has the potential for major anti-inflammatory effects. The precise mechanism whereby MKP-1 is induced by flow is currently unknown.
In summary, prolonged flow establishes a quiescent endothelial phenotype by the following redundant and somewhat interacting mechanisms: (1) use of the initial pro-oxidant burst as a trigger for the induction of cellular antioxidant defenses; (2) capitalizing on the reduction of ROS to downregulate JNK (through decreased TXNIP and increased Trx) and NF-κB signaling; (3) activation of Akt/PKB, resulting in strong eNOS upregulation and antiapoptotic signals as well as diversion of NF-κB to a largely anti-inflammatory role with further support of eNOS induction; (4) greatly upregulated NO production, resulting in inhibition of NF-κB inflammatory signaling, downregulation of NADPH oxidase activity, and decreased expression of the AT1R as well as other protective activities; and (5) MAPK pathway crosstalk and induction of MKP-1, leading to inhibition of p38 and JNK signaling while promoting signaling by protective MEK5, ERK5, and, most important, KLF2.
Genomic Studies of Flow-Mediated Change in Endothelial Phenotype
Several groups have examined RNA expression profiles of endothelial cells exposed to the atherosclerosis-prone versus protective flow patterns. In these studies, expression of up to 10,000 or more genes could be assessed simultaneously by examining differences in the levels of mRNA found in harvested endothelial cells exposed in vitro to the different flow patterns. Although there were a number of differences in technical approaches used in these studies and differences in specific findings of which genes were significantly upregulated or downregulated, this genome-wide approach generally confirmed the findings of candidate gene and molecular studies in which genes associated with inflammation and susceptibility to atherosclerosis tended to be increased in cells exposed to the slow-flow, oscillatory pattern, whereas such genes were suppressed in the cells exposed to rapid, unidirectional pulsatile flow.
In one of the studies, endothelial cells were harvested from atherosclerosis-prone and atherosclerosis-protected areas of aorta from normal pigs. This in vivo approach suggested that whereas proinflammatory genes were frequently increased in the atherosclerosis-prone areas, some potentially compensating genes were also upregulated, including several antioxidant genes, such as glutathione peroxidase. Also, there seemed to be little evidence of frank inflammation in these arteries. Thus, active NF-κB was not found in greater levels in nuclei, nor were several key adhesion receptors found to be expressed at higher levels on the cell surface. The authors interpreted these results to suggest that disrupted or slow flow primes the endothelium to respond with an enhanced inflammatory response if it is exposed to additional risk factors. Thus, “a delicate balance of pro- and antiatherosclerotic mechanisms may exist simultaneously in endothelium of lesion-prone sites of the aorta to create a setting of net vulnerability to atherogenesis, but with protective measures also present. A shift from athero-susceptibility to atherogenesis may occur by inhibition of the protection.”
This concept of predisposition is supported by the finding of an increase in both bound NF-κB and its inhibitor IκB in the cytoplasm of endothelial cells from atherosclerosis-prone areas of the aorta in mice but with no increase of NF-κB in the nucleus. Nevertheless, there was much greater activation of NF-κB and its inducible gene products in these same hemodynamically prone areas after the mice were treated with lipopolysaccharide or after feeding LDL receptor (LDLR)–deficient mice a high-fat diet to induce hyperlipidemia.
Further Endothelial Activation by Dyslipidemia
The well-known observation of monocyte adherence to endothelium in atherosclerosis-prone areas just days after induction of severe hypercholesterolemia by cholesterol feeding in animals can be better appreciated in light of the above molecular mechanisms. Factors that even modestly activate endothelial cells would be expected to initiate substantially greater effects in the already “primed” cells found in atherosclerosis-prone areas. Hyperlipidemia clearly provides one or more signals for such activation, whereas lowering of serum cholesterol, even if only by dietary means, clearly decreases endothelial activation. As early as 2 hours after injection of human LDL into rabbits, grapelike clusters of aggregated LDL could be seen enmeshed in focal areas of the subendothelial matrix. VCAM-1 and MCP-1 are expressed by endothelial cells within at least 3 weeks of starting of a high-cholesterol diet in rabbits. Not only do lipoproteins preferentially accumulate in atherosclerosis-prone areas, but hyperlipidemia itself clearly increases the permeability of the endothelium and total area of susceptibility, suggesting direct activation. Both activation of endothelium with increased cell turnover and diminished glycocalyx height appear to mediate this increased permeability due to hyperlipidemia. This appeared true with even modest hyperlipidemia as newly synthesized, radiolabeled thymidine (an index of cell turnover) in aortic endothelial cells tripled in just 3 days after starting of a high-cholesterol diet in pigs when serum cholesterol levels had increased to only 187 mg/dL (normal, 74 mg/dL). Thus, hyperlipidemia clearly activates endothelium, but the precise signals mediating this effect have been less easily identified. Given the current evidence, it would appear that multiple mechanisms linking hyperlipidemia to endothelial activation are likely, further illustrating the principle of redundancy. Several of these possible mechanisms are presented briefly here.
LDL Oxidation
Much evidence has been forwarded in support of the LDL oxidation hypothesis for endothelial activation (and still more for foam cell formation, to be discussed later). Native LDL appears to accumulate in the intima of human fetuses before macrophages are found. Appearance of oxidized LDL seems to follow native LDL, and the oxidized LDL are frequently present without macrophages nearby. However, the most common finding was the presence of intimal macrophages together with oxidized LDL ( Fig. 8-8 ). Severely oxidized LDL (oxLDL) have been shown to stimulate adhesion of both macrophages and T cells to endothelial cells, to promote diapedesis into the subendothelium, and to lead to the arrest of egress. Whereas LDL bearing oxidation-related epitopes were seen frequently when macrophages were present, even in fetal aorta (see Fig. 8-8 ), the more severely oxidized oxLDL are generally found in advanced lesions primarily in association with macrophages. Such oxLDL are thought to be more likely formed by exposure to myeloperoxidase-produced hypochlorous acid from activated macrophages. This consideration raises the question of whether oxLDL help summon the macrophages initially or whether oxLDL are formed only after activated macrophages have arrived. Nevertheless, even if formation of oxLDL or chlorinated and nitrosylated forms of LDL require preexisting macrophages, such modified LDL could subsequently perpetuate endothelial activation and otherwise promote atherosclerosis. One important issue to note in this regard is the near absence of myeloperoxidase in experimental mouse atherosclerotic lesions, suggesting a major species difference with humans and providing a likely explanation for the lack of effect on murine atherosclerosis after knockout of myeloperoxidase, whereas humans with myeloperoxidase deficiency appear to be protected from atherosclerosis. Perhaps the strongest evidence in favor of the oxidized LDL hypothesis comes from the decrease in atherosclerosis seen after immunization of animals with various forms of oxidized LDL or malondialdehyde-treated LDL. Still, the immunization appeared to be most effective at later stages of atherosclerosis. Given these considerations, there remains the need to explain how the endothelium is activated to attract the monocytes in the first place.

Aside from oxLDL (usually produced in vitro with nonphysiologic exposure to copper or iron ions), endothelial and smooth muscle cells can mildly oxidize LDL in culture, and such “minimally modified” LDL (mmLDL) can also activate endothelial cells and initiate monocyte adherence and transmigration. These events were prevented by the presence of high-density lipoproteins (HDL) or antioxidants. In these studies, only monocyte, not neutrophil, adhesion and migration was stimulated, much as might be expected for atherosclerosis-related endothelial activation. Presumably, LDL trapped in the subendothelial space would be exposed to sufficient ROS from activated endothelial cells to result in such minimally oxidized LDL. Formation of mmLDL or oxLDL in the plasma is thought to be unlikely because of potent antioxidant factors there. Some have questioned whether even mmLDL are formed in vivo in the surprisingly antioxidant-rich intima. However, the observations in human fetuses cited before would suggest that at least some oxidation is possible and occurs before the arrival of macrophages.
mmLDL and Toll-Like Receptor 4 (TLR4)
Perhaps the strongest evidence for a pathway mediating endothelial activation by mmLDL is by way of toll-like receptor 4 (TLR4). TLR4 is a major mediator of innate immunity and the main response receptor for bacterial lipopolysaccharide. A number of pathogen-associated molecular patterns (PAMPs) appear to trigger inflammatory responses through TLRs. Oxidized or otherwise altered phospholipids protrude abnormally (much like whiskers) from the cell membrane and trigger innate immune receptors and natural antibodies that act to clear the damaged phospholipids. These considerations may be most relevant to macrophage activation and foam cell formation, considered later. Nevertheless, endothelial cells from C3H/HeJ mice, which have an inbred mutation in TLR4, are essentially unresponsive to mmLDL and are protected from diet-induced atherosclerosis. Furthermore, mmLDL as well as associated oxidized phospholipids were shown to transmit signals to activate NF-κB through TLR4. Knockout of TLR4 or its downstream adapter protein MyD88 (myeloid differentiation factor 88) resulted in unresponsiveness to mmLDL in endothelial cells and decreased atherosclerosis. As noted later, TLR4 may also be involved in other mechanisms of endothelial activation in relation to atherosclerosis. Several features of TLR4 signaling are shown in Figure 8-9 . TLR2 may also be involved in endothelial activation.

Role of the Oxidized LDL Receptor 1 (LOX-1)
Early studies with oxLDL demonstrated powerful proinflammatory and apoptotic effects on incubation with endothelial cells. Subsequent studies identified LOX-1 (lectin-like oxidized LDL receptor 1), coded by the OLR1 gene (oxidized LDL receptor 1), as a scavenger-type receptor expressed on the surface of endothelial cells that likely mediated these effects. LOX-1 is also expressed on monocyte-macrophages and platelets, but unlike macrophages, LOX-1 is the only scavenger-type receptor expressed highly on endothelial cells. LOX-1 may be considered another PAMP-recognizing receptor with a key role in innate immunity. Importantly, LOX-1 is not expressed constitutively but is induced by most of its ligands, including oxLDL, as well as by factors that upregulate NADPH oxidase, such as angiotensin II. Besides oxLDL, LOX-1 is bound and activated by gram-positive and gram-negative bacteria, apoptotic bodies, senescent red blood cells, activated white blood cells and platelets, advanced glycation end products (AGEs), lysolecithin, and, recently recognized, C-reactive protein. LOX-1 is expressed particularly in atherosclerosis-prone areas or over atherosclerotic plaque and has been reported to be increased by hyperlipidemia, diabetes, and hypertension and after exposure to various cytokines.
Details of LOX-1–mediated intracellular signaling continue to be worked out. Some of the identified pathways are depicted in Figure 8-9 . Intracellular signaling pathways activated by LOX-1 include RhoA (resulting in eNOS downregulation); a Rac1-mediated burst in NADPH oxidase activity ; the Ras, Raf, ERK1/2 cascade, leading to increased PAI-1 expression ; and increased expression of CD40 and CD40L (CD40 ligand) through PKCα signaling, which may act in an autocrine fashion through CD40L signaling to further upregulate several inflammatory genes including TNF-α and P-selectin. Importantly, activation of LOX-1 leads to inactivation of PI3K. In endothelial cells, PI3K is strongly protective as it normally upregulates eNOS and inhibits apoptosis. Upregulation of PI3K is key to the protective autoregulation by fibroblast growth factor 2 (FGF2) through its own receptor, FGFR2, as shown in Figure 8-9 . Therefore, by blocking of PI3K and through increased TNF-α production, apoptosis is greatly accelerated.
If oxLDL were the only lipoprotein to bind to the LOX-1 receptor, its relevance to early endothelial activation would not be so readily apparent for reasons noted before. However, studies note that in addition to oxLDL, LOX-1 is bound and strongly activated by electronegative LDL. This finding is consistent with a substantial literature demonstrating the endothelial activating and apoptotic potential of electronegative LDL. These particles can be formed by a number of nonoxidative mechanisms as well as by oxidation, including glycation, enrichment with nonesterified fatty acids, treatment with cholesteryl esterases, phospholipase A 2 , platelet-activating factor acyl hydrolase (PAF-AH, also called LpPLA 2 ), and, importantly, sphingomyelinase. Electronegative LDL are found in a much higher proportion of plasma LDL than are oxidized LDL (up to 10% of normolipidemic LDL). Recently, evidence was presented that LDL themselves carry a sphingomyelinase activity that promotes formation of electronegative LDL and that promotes LDL aggregation as seen in subendothelial, trapped LDL. Further, sphingomyelinase and other enzymes capable of modifying LDL and promoting aggregation are present in the artery wall and likely promote aggregation of LDL. Importantly, other studies identify LOX-1 as a receptor that can mediate inflammatory responses to TGRL (triglyceride-rich lipoprotein) remnants.
Particles that bind to LOX-1 appear to be relevant in vivo because overexpression of LOX-1 in the liver to remove such particles from plasma led to virtual arrest of atherosclerosis progression. Knockout of LOX-1 reduced atherosclerosis approximately 50% in a high-cholesterol/fat–fed LDLR knockout model and preserved endothelial function. Overexpression of LOX-1 in coronary arteries in apo E knockout mice leads to an atherosclerosis-like vasculopathy.
TGRL remnants may be particularly atherogenic. In one study, TGRL appeared to activate endothelial cells without any need for modification. In another study, apo C-III, which accumulates on TGRL, appeared to activate PKCß and thereby inhibit protective Akt signaling in endothelial cells. In a gene expression study comparing VLDL with oxidized VLDL (oxVLDL), different endothelial pathways were activated. Thus, native VLDL upregulated ERK1/2 and NF-κB modestly with little effect on ROS generation, whereas oxVLDL resulted in substantial stimulation of the MAPK p38, NF-κB, and marked increase in ROS production as well as evidence for decreased viability. Later studies by this same group showed much greater endothelial activation by postprandial TGRL after a cream meal compared with fasting TGRL from hypertriglyceridemic patients. In addition, flow-mediated dilation of the brachial artery was impaired by the fatty meal. Postprandial TGRL resulted in upregulation of p38 MAPK, CREB, NF-AT, NF-κB, VCAM-1, PECAM-1, ELAM-1, ICAM-1, P-selectin, MCP-1, IL-6, TLR4, CD40, ADAMTS1, and PAI-1. The receptors or other mechanisms mediating these responses were not identified.
RAGE
The receptor for advanced glycation end products (RAGE) is present on endothelial cells and, like TLR4 and LOX-1, can activate endothelial cells by overlapping intracellular signaling pathways (see Fig. 8-9 ). JNK activation seems to be particularly important in this regard, but RAGE also activates the JAK-STAT3 pathway. RAGE is generally considered in the context of diabetes-related modifications to proteins or LDL. Indeed, it recognizes a ligand generated by nonenzymatic reactions between glucose and protein lysine residues (carboxy-methyl lysine). Certainly, ligation of RAGE by this ligand, generated in proportion to degree of hyperglycemia, is one means whereby diabetes contributes to endothelial activation and atherosclerosis, as demonstrated by use of soluble RAGE competition as well as diabetic RAGE knockout models. However, hyperlipidemia without diabetes also generates a substantial load of ligands for the RAGE. Indeed, 52% reduction in atherosclerosis was seen in nondiabetic, apo E knockout mice that were also deficient in RAGE, whereas those expressing endothelial cell–specific dominant-negative RAGE mutations had more than 70% reduction. Ligands for RAGE present in oxLDL identified in this model included proinflammatory S100/calgranulins and HMGB1 (high-mobility group box 1). Incubation with S100 activated JNK with elaboration of VCAM-1 in cultured wild-type endothelial cells, whereas activation was substantially reduced in the RAGE knockout or dominant-negative cells. Thus, RAGE represents yet another pathway for endothelial cell activation.
Reflections on Endothelial Activation
One of the major messages in these discussions is the redundancy of pathways potentially leading to endothelial cell activation. For modified lipoproteins alone, activation may occur by way of TLR4, LOX-1, or RAGE. The TLR4 and RAGE responses may require oxidative changes, as found in mmLDL or oxidized phospholipids, but may also be triggered by saturated fatty acids (for TLR4); the LOX-1 response appears to include a broader array of triggers. Other potential pathways resulting in endothelial activation from various lipoprotein moieties are also likely. Thus, an intervention that focuses on only one of these pathways, such as oral antioxidants (even acknowledging the limitations of the antioxidants tested thus far), might be expected to be ineffectual in reducing coronary events. This can be expected even if oxidation is causally related to disease because of the redundancy of lipoprotein modifications that can lead to endothelial activation. For the same reason, effects from single genes in these pathways may be difficult to detect. Indeed, not shown in Figure 8-9 are the numerous cytokine-mediated pathways that lead to activation. Additional pathways are illustrated by numerous knockout models reviewed in Tables 8-1 and 8-2 .
Endothelium | Effect | Leukocyte | Effect |
---|---|---|---|
Tethering or Rolling | |||
E-selectin | ↓ | ESL-1, CD44 | ↓↓ |
P-selectin | ↓ | PSGL-1 | ↓ |
PSGL-1, CD24, CD34 | ↓ | L-selectin | — |
Activation by Chemokines/Chemoattractants | |||
M-CSF | ↓↓↓ | CSF1R | ↓↓ |
MCP-1 (CCL2) | ↓↓ to ↓↓↓ | CCR2 | ↓↓ to ↓↓↓ |
CCL5 (RANTES) | ↓ to ↓↓ | CCR1 CCR5 | ↑↑ ↓ |
CXCL1 (GROα) | ↓ | CXCR2 (BMT) | ↓↓ |
CXCL2 (GROβ) CXCL3 (GROγ) CXCL5-7 | — | CXCR2 | ↓↓ |
CXCL4 (PF 4) | ↓↓ | CXCR3B | — |
CXCL8 (IL-8) | — | CXCR2 | ↓↓ |
CXCL9, 10, 11 | ↓ | CXCR3 | NS |
CXCL12 (SDF-1) | — | CXCR4 | ↑ |
CXCL16 | ↑ | CXCR6 | ↓ |
CX3CL1 (fractalkine) | ↓↓ | CX3CR1 | ↓↓ |
CD40 | NS | CD40L (CD154) | ↓↓↓ |
Slow Rolling and Firm Adhesion | |||
VCAM-1 | ↓ | α 4 β 1 integrin (VLA4) | — |
Fibronectin | ↓↓ | α 4 β 1 , α 5 β 1 | — |
ICAM-1 | NS to ↓↓ | α L β 2 integrin (LFA1) | ↓ |
E-selectin | ↓ | α M β 2 integrin | — |
vWF | — | α IIb β 3 integrin | — |
Thrombospondin-1 (VN) | — | α V β 3 integrin | ↑↑ |
MADCAM1 | — | α 4 β 7 integrin | — |
CD137 | — | CD137L | — |
Transmigration | |||
JAM-A | — | LFA1, JAM-A | — |
JAM-B | — | VLA4, JAM-C | — |
JAM-C (also in smooth muscle cells) | — | MAC1, JAM-B | — |
CD99 | ↓ | CD99 | — |
PECAM-1 | ↓ to ↓↓ | PECAM-1 | ↓ to ↓↓ |
ICAM-2 | — | MAC1, LFA1 | — |
* In most cases, monocyte interactions are shown. Interactions with other leukocytes and platelets may follow similar steps. Cells other than endothelial cells may produce the chemokines shown.
Redundancy seems to be the rule for pathways vital to the survival of the organism. In the case of endothelial activation, the key role such activation plays in defense from microorganisms as well as in clearing the bloodstream of senescent or apoptotic debris seems evident. In this sense, it may be disingenuous to describe the state of endothelial activation associated with various risk factors as “dysfunction.” Indeed, the cells seem to be performing admirably and as expected in response to perceived threats.
Molecular Biology of Leukocyte Transmigration into Intima
The first step of capturing passing leukocytes has generally been considered to be mediated by endothelial cells expressing on their surface the adhesion molecules P-selectin and E-selectin. Binding of these selectins to their cognate receptors on leukocytes is relatively loose, with breaking and reforming of the bonds, thereby leading to partial tethering and rolling of leukocytes under the shear forces of the blood circulation. L-selectin on virtually all leukocytes can recognize several markers on activated endothelial cells as well. Tethering and rolling bring the leukocyte into intimate contact with the endothelial surface, where the leukocyte encounters chemotactic cytokines or chemokines such as MCP-1 (also referred to as CCL2) and monocyte colony-stimulating factor (M-CSF) as well as other surface-immobilized chemokines that are held close to the endothelial surface by proteoglycans in the glycocalyx. More than 40 chemokines are known, again illustrating the redundancy of key systems.
The current terminology for the chemokines refers to characteristic conserved cysteine residues in the N terminus. These cysteines can have no intervening amino acids (CC chemokines), one separating amino acid (CXC chemokines), or three intervening amino acids (CXXXC or CX3C chemokines); L refers to the chemokine ligand and R to the receptor. For example, some of surface-immobilized chemokines noted before include CXCL1, CXCL2, CXCL4, and CCL5 in addition to MCP-1 (CCL2). Chemokines presented by activated endothelial cells bind to cognate receptors that are all GPCRs (such as CCR2, the receptor for MCP-1) located on the surface of the leukocyte. This binding leads to further activation. At least 20 such receptors are known. Even before this step, rolling appears to promote movement of these GPCRs from intracellular sites to the cell surface in association with cholesterol-rich rafts. One study demonstrated the presence of MCP-1, GROα, and IL-8 on the endothelium of human atherosclerotic plaque that was capable of inducing attachment and spreading of test monocytes, suggesting the relevance of prior, mostly mouse-based models and further illustrating redundancy in this early step of atherogenesis.
Binding of chemokines to their cognate GPCRs initiates “inside-out signaling,” with the result being an alteration in the extracellular configuration of leukocyte integrins leading to enhanced binding affinity to endothelial VCAM-1 and ICAM-1 (or other ligands). This enhanced interaction then leads to slow rolling, adhesion strengthening, spreading, and firm adhesion. The firm binding of leukocyte integrins with their ligands then initiates “outside-in signaling” and clustering of the integrins into focal adhesions. Outside-in signaling refers to a host of integrated signals initiated by the bound integrins that result in intraluminal crawling as the leukocyte seeks an opportune site for penetration and finally diapedesis or transmigration of the leukocyte into the subendothelium. This may occur by passage of the leukocyte between endothelial cells by interactions with various gap junction proteins (paracellular migration) or directly through thinned segments of endothelial cells (transcellular migration). For monocytes, this is followed by transformation into tissue macrophages or dendritic cells. There are at least 24 different integrin heterodimers that mediate this signaling on various leukocytes.
Each progressive step of this process is associated with greater activation of the leukocyte and coordinated intracellular and intercellular signaling with endothelial cells. Whereas monocyte transmigration has been considered to predominate in atherosclerotic lesion formation, the participation of other leukocytes and subsets of monocytes and T cells has recently been more fully recognized. Platelets can also adhere to activated endothelial cells and may aid in binding of other white cells. T cells, mast cells, and even a few B cells and neutrophils also find their way into the incipient lesion. Once they are in the subendothelial space, there is an exchange of cytokines between the various activated cells that further amplifies the inflammatory response. The molecular biology of these steps in relation to atherosclerosis has been extensively studied and is the focus of several recent, excellent reviews. Some of the ligands and receptors mediating these endothelial cell–leukocyte interactions are listed in Table 8-2 . However, virtually any presentation of leukocyte activation and interaction with the endothelium must be considered a simplification. One review listed 47 proteins that were thought to be regulatory for at least 900 total proteins and 6000 protein-protein interactions. Gene expression profiling identified 400 genes that were upregulated or downregulated by at least twofold after exposure of endothelial cells to IL-1β, IFN-γ, and TNF-α. About 600 to 1000 genes were similarly regulated during the transition of monocytes to macrophages. Only a few additional details of some of the key processes are provided here.
As noted before, initial tethering and rolling of leukocytes are mediated, in part, by P-selectin and E-selectin on activated endothelial cells and L-selectin on leukocytes. PSGL1 (P-selectin glycoprotein ligand 1) can bind all these selectins. Other ligands can also bind E-selectin. Nonactivated integrins can bind weakly to VCAM-1 and ICAM-1 and can also mediate rolling. However, activated integrins are generally considered more important in leukocyte firm adhesion and spreading. P-selectin is also expressed on activated platelets. P-selectin is presynthesized and stored in Weibel-Palade bodies and can be expressed on the cell surface rapidly by fusion of the Weibel-Palade body with the cell membrane. In contrast, E-selectin is not stored and must be synthesized de novo; thus, levels rise more slowly on activation.
The early recognition of the predominance of macrophages in human and experimental plaques focused most attention on the early entrance of these cells into the intimal space. However, the importance of other cells has been increasingly recognized. Further stimulation of macrophages or dendritic cells by Th1 cells as well as mutual stimulation by macrophages and dendritic cells seems to establish inflammatory cells within the intima as long as any inciting risk factors (such as hyperlipidemia) are present. Neutrophils may become particularly important in destabilizing advanced lesions.
In a startling set of experiments, an extensive network of dendritic cells were found to be the earliest inhabitants of this space, concentrated clearly in atherosclerosis-prone areas of disturbed flow. This network was established in all specimens examined even in infancy or early childhood in the absence of any risk factors, well before the presence of any evidence of macrophage accumulation or lipid-filled cells, and was universally present in normolipidemic animals as well. The participation of these cells in addition to early appearance of proinflammatory Th1 lymphocytes, which may respond to the self antigen HSP-60, together with the observation that patients with CAD were found to have increased plasma titers of anti–HSP-60 antibodies that strongly cross-reacted to certain Cytomegalovirus antigens has raised considerable interest. Evidence has been presented that immunization of LDLR knockout mice with the bacterial homologue HSP-65, HSP-60, or desensitizing peptide fragments shifts lymphocyte counts to greater numbers of regulatory Th2 (anti-inflammatory) in relation to Th1 cells with increased IL-10 production and substantially reduces atherosclerosis by 50% to as much as 80%. Whether such desensitization therapy may be effective to prevent progression of human atherosclerosis is unknown.
Because movement of leukocytes has been so extensively studied in the context of many diseases, the elegant mechanisms controlling their movement and chemotaxis will not be discussed in detail. It is recommended that the interested reader consult recent textbooks for the elegant coordination of these events, in which GTPase molecules Cdc42 and Rac serve as both controlling “switches” and membrane attachment points for growing and branching actin microfibers that provide a pushing force to propel the cell forward, while Rho works at the back of the cell to promote uropod retraction. Kinesin motors serve to recycle integrins and other key cell machinery forward while other signaling mechanisms maintain cell polarity.
Once early inflammatory changes have been established, particularly with recruitment of the various leukocytes to the subendothelial space, numerous cytokines are secreted by activated cells that reinforce the inflammatory response and further activate endothelial cells as well as surrounding leukocytes. This exchange of cytokines acts as a positive feedback loop to ensure a vigorous response to perceived threats. In Table 8-3 , a list is provided of some of these cytokines as well as other genes affecting early stages of endothelial activation and inflammation and effects on atherosclerosis of genetic manipulation. The receptors and intracellular signaling in response to these cytokines have been the subject of much study, and many illustrations of these pathways are available for free download from commercial sources on the Internet (e.g., http://www.sabiosciences.com/pathwaycentral.php and http://www.cellsignal.com/reference/pathway/index.html ) as well as a recent on-line textbook of cell signaling pathways ( http://www.cellsignallingbiology.org.easyaccess1.lib.cuhk.edu.hk/ ). They will not be discussed further here.
Gene | Effect |
---|---|
Secreted by Macrophages in response to Toll Receptor and Scavenger Receptor Binding | |
IL-1α | ↓↓ |
IL-1β | ↓ |
IL-6 | NS |
gp130 (IL6ST, in humans) | ↓ |
IL-12 | ↓↓ |
IL-18 | ↓ |
TNF-α | ↓↓ |
MIF | ↓↓ |
Secreted primarily by Th1 cells after Activation by Antigen Presentation (Dendritic Cell or Macrophage), Reinforced by CD40-CD40L Costimulation | |
IL-2 | ↓ |
IFN-γ | ↓ to ↓↓ |
Secreted primarily by Th2 cells after Activation by Antigen Presentation (Primarily Anti-Inflammatory) | |
IL-4 | NS |
IL-5 | ↑ |
IL-10 | ↑ |
Secreted primarily by T Regulatory Cells | |
TGF-β | ↑↑ |
Secreted by Mast Cells | |
GM-CSF | ↓ to ↑ |
Miscellaneous | |
IL-1Ra (TG) | ↓ |
IL-1R | ↓↓↓ |
TNFR1 (p55) | ↑↑ |
IFN-γR | ↓↓ |
While reviewing Tables 8-1 through 8-3 , the reader should keep in mind that the apparent impact on atherosclerosis of manipulating a given gene can change over time. Most of these models are double knockouts; the first knockout (of the apo E gene or the LDL receptor) results in hyperlipidemia, and the second knockout is of the test gene under study. Short-term studies generally report a greater percentage reduction in atherosclerosis due to test gene knockout than longer term studies do. For example, in one study, at 5 weeks there was a clear effect of even heterozygous CCR2 (the receptor for MCP-1) knockout, with atherosclerosis clearly increasing progressively from CCR2 −/− (strongly protected) to CCR2 +/− to CCR2 +/+ (wild type). By 9 weeks, however, the heterozygous knockout had caught up to the wild type. By 13 weeks, even the homozygous knockout mice had atherosclerosis extent similar to that for wild type at 9 weeks, although the trajectories for atherosclerosis remained different between CCR2 −/− and CCR2 +/− mice.
What do such observations imply for gene effects in human atherosclerosis, a disease that develops over decades? Even with powerful (but presumably rare) effects on a clearly important gene, there may be no observable effect in the long run in a redundant system. This principle may help explain the extreme difficulty that human gene hunters have had in identifying consistently replicable genetic associations with atherosclerosis, particularly involving initiating pathways (endothelial activation and inflammation). A corollary may be that interventions that have global impact on such pathways (such as control of hyperlipidemia or a reduction in blood pressure) will likely have more success than efforts to affect a single genetic element. An additional consideration with regard to inflammatory pathways is that a focused intervention such as that noted for CCR2 knockout that resulted in marked reductions in monocytes may be associated with unacceptable increases in susceptibility to infection.
Effects of Selected Risk Factors on Initiation of Atherosclerosis
Blood Pressure
Pressure, stretch, and flow are the first risk factors to which the endothelium is exposed. Blood pressure in the arterial range is an essential requirement for the development of atherosclerosis. Venous atherosclerosis does not occur even in patients with homozygous familial hypercholesterolemia. The relatively rapid progression of atherosclerosis in saphenous veins used in coronary artery bypass suggests that there is nothing uniquely resistant about veins themselves. Normally, the pulmonary arterial circulation with its systolic pressures of 12 to 22 mm Hg is another entirely protected site. Nevertheless, with pulmonary hypertension, atherosclerotic plaques are commonly seen.
The major mechanism whereby pressure promotes atherosclerosis appears to be through increased pressure-driven convection of LDL and other lipoproteins into the intima. For example, LDL accumulation in the intima of pressurized rabbit aorta increased 44-fold when pressure was raised from 70 to 160 mm Hg. Stretch may also play a role. Approximately 90% of the convection of LDL into the subendothelial space appears to be through gaps created between mitotic endothelial cells in areas of low shear stress, whereas only 10% was estimated to enter by way of transcellular vesicular traffic. A thinner glycocalyx, also associated with atherosclerosis-prone areas with low shear stress, also appears to contribute to greater LDL permeability.
Exercise
Even modest exercise serves as a potent stimulus to improved endothelial function as measured by vasodilation in response to acetylcholine, particularly in older animals and humans. Aged mice had much higher levels of nitrotyrosine (a marker of chronic oxidative stress) in their aortas compared with younger mice, and this was markedly reduced by modest amounts of voluntary running on an exercise wheel. Exercise acutely increases flow and hence shear stress experienced by endothelial cells in many vascular beds, not just the exercising limb. This increased flow is sensed by multiple transduction mechanisms, several of which acutely stimulate NO production as well as pro-oxidant systems. NO can acutely block several pro-oxidant pathways and inflammatory pathways, including direct inhibition of NADPH oxidase and IKK. Further, NO alters transcription of eNOS and leads to increased expression of eNOS protein. Thus, inhibition of eNOS activity abrogates the exercise-induced increase in eNOS protein expression.
Stimulation of flow has a number of additional effects. An acute increase in flow with exercise results in a transient increase in endothelial superoxide anion generation by mitochondria and NADPH oxidase. This superoxide is converted rapidly to hydrogen peroxide. The modest, controlled burst of hydrogen peroxide, in turn, also acts to promote increased expression and activity of eNOS, possibly (as noted before) through altered expression of NF-κB as well as stimulation of HSP-90 (which strongly supports eNOS activity) through stimulation of HNF1. Importantly, when high levels of human catalase were expressed in endothelial cells of exercising mice, blocking signaling by hydrogen peroxide, the expected increase in eNOS expression in response to exercise was entirely blocked.
Endothelial cells also adapt to chronic laminar flow by stimulation of a number of antioxidant defenses (at least in part through Nrf2 signaling), resulting in increased expression of extracellular superoxide dismutase and Cu/Zn SOD as well as a decrease in NADPH oxidase. Similar adaptive changes occur with exercise training, explaining the longer term antioxidant effects of exercise despite acute modest stimulation of superoxide and hydrogen peroxide production during acute bouts of exercise. Parallel benefits from exercise on insulin signaling pathways in skeletal muscle appear to be blocked by large doses of oral antioxidants, suggesting potential deleterious effects of such interventions. Finally, exercise was shown to clearly reduce atherosclerosis in apo E–deficient mice together with marked reduction of macrophage and Th1 cell accumulation in the intima. This effect of exercise was entirely blocked by inhibition of eNOS. Other effects of exercise on endothelial function, including increased PGI 2 , thrombomodulin, and plasmin, have also been reviewed.
Hyperlipidemia
Extensive effects of hyperlipidemia to initiate atherosclerosis are reviewed earlier. Not only does hyperlipidemia activate the endothelium and otherwise promote atherosclerosis, but effects on circulating white cells are also apparent. Thus, expression of CCR2, the cognate receptor on monocytes for MCP-1, was approximately twofold higher in hypercholesterolemic patients with average LDL of 167 mg/dL compared with persons with LDL of 80 mg/dL. Incubation of monocytes in hypercholesterolemic serum, without LDL oxidation, led to similar marked increases of CCR2, apparently through uptake by the LDL receptor. Additional unexpected effects of hypercholesterolemia include suppression of the inward rectifying potassium current in endothelial cells (which might be expected to lead to diminished NO response to acute changes in flow). Hypercholesterolemia affects phosphatidylinositol 4,5-bisphosphate (PIP 2 )–sensing amino acids in inwardly rectifying K (Kir2) channels, not by altering membrane physical properties. Interestingly, patients with familial hypercholesterolemia were shown to have reduced glycocalyx volume, which could be partially restored by treatment with rosuvastatin.
Hypercholesterolemia and particularly hypertriglyceridemia appear to increase activity of xanthine oxidoreductase (XOR). There may be both greater conversion in the liver of the enzyme from the dehydrogenase form to the oxidase form (accompanied by greater production of superoxide) and increased release of the oxidase from the liver. Xanthine oxidase is then thought to adhere to the glycocalyx of endothelial cells, where it can contribute to endothelial oxidative stress and promote endothelial dysfunction that may be ameliorated by XOR inhibitors such as allopurinol. XOR knockout studies are not feasible in mice as the pups are stunted and live only 4 to 6 weeks, presumably because of renal failure. Nevertheless, tungsten administration (a relatively specific inhibitor of XOR) to apo E–deficient mice fed a Western-type diet for 6 months resulted in 83% reduction of aortic atherosclerosis.
HDL Effects
Besides reverse cholesterol transport, HDL has been shown to mediate a number of protective effects on the endothelium. HDL protects endothelial cells from apoptosis induced by oxLDL, TGRL, TNF-α, and activated complement. HDL stimulates NO production by eNOS and increases prostacyclin (PGI 2 ) production through COX-2. Finally, HDL suppresses expression of VCAM-1, ICAM-1, and E-selectin by endothelial cells as well as other signs of endothelial activation after exposure to oxLDL or cytokines. These effects are mediated by both apo A-I and lipid components carried in HDL, particularly sphingosine 1-phosphate (S1P) and related lysosphingolipids.
There are five known S1P receptors, S1P1 through 5. These are G protein–coupled receptors (GPCR) with the potential to be coupled to a variety of heterotrimeric G proteins with diverse and frequently divergent functions. The atheroprotective functions of HDL on endothelial cells appear to be mediated by S1P signaling primarily through the S1P1 receptor and to a lesser extent through S1P3. HDL carries more than 50% of the S1P in plasma. Activation of the S1P1 receptor recapitulates a number of the protective features of prolonged exposure to laminar flow (see Fig. 8-7 ). These effects appear to be mediated by ERK1/2, PI3K, Akt, and Rac1 activation, with resulting increased barrier integrity, increased NO production, suppression of inflammatory responses, promotion of cellular antioxidants, and increased cell survival (by suppression of caspases). Activation of these pathways is supported by and possibly dependent on the binding of apo A-I to endothelial SR-B1. Binding to SR-B1 not only brings the HDL into proximity with S1P1 receptors but also supports cytosolic signaling to activate Src. Src can then activate MEK1/2 followed by ERK1/2 as well as PI3K. PDZK1 is an adaptor or scaffolding protein that binds to the cytosolic side of SR-B1. PDZK1 is required for Src activation by HDL. Indeed, PDZK1 knockout completely blocked HDL-mediated increases in eNOS and promotion of endothelial repair after injury. HDL may also inhibit monocyte activation after interactions with T cells.
Another mechanism whereby HDL protects endothelial cells and promotes NO production is by removal of the oxysterol 7-keto cholesterol by way of the ABCG1 transporter. This oxidized sterol built up when mice were fed a Western-type diet. HDL also helps prevent LDL oxidation or detoxifies oxidized phospholipids in LDL through the HDL-associated enzyme PON1. PON1 knockout mice express increased atherosclerosis, whereas overexpression of PON1 was protective. These findings contrast with the much more variable outcomes associated with milder effects on PON1 caused by common human variants.
Diabetes
Whereas other risk factors may recapitulate a form of endothelial activation that in some settings would be advantageous (such as in infection or physical injury), the endothelial response in diabetes is truly maladaptive and finds few if any parallels in normal physiology. Therefore, the term endothelial dysfunction applies most aptly in diabetes. Unlike other risk factors, hyperglycemia leads to endothelial dysfunction that is systemic, resulting in both aggravation of atherosclerosis and a microvascular disease that is unique to diabetes. In type 2 diabetes, excess free fatty acid exposure and insulin resistance appear to further exacerbate this response.
An elegant, unified model to explain the seemingly disparate aspects of endothelial dysfunction in diabetes has been forwarded. The endothelial cell is one of a handful of cell types that are unable to regulate entry of glucose into their cytoplasm. Thus, hyperglycemia leads to unregulated uptake of glucose through GLUT1 transporters and elevated intracellular glucose in endothelial cells. Rapid flux of substrate through the glycolytic pathway then ensues, leading to an abundance of pyruvate for transfer into mitochondria, synthesis of acetyl coenzyme A, and generation of reducing equivalents (NADH, FADH 2 ) by the citric acid cycle. The transfer of high-energy electrons from NADH and FADH 2 to complexes of the electron transport chain provides energy to pump hydrogen ions from the matrix into the mitochondrial intermembrane space, thereby generating the hydrogen ion gradient that subsequently drives ATP synthesis through F 0 F 1 complexes. With an overabundance of energy substrate, the hydrogen gradient increases to a critical level, at which point the final electron transport to complex IV is impaired (where water is normally formed from controlled electron transport to oxygen). The “backed up” electrons then begin to be transported from coenzyme Q 10 (ubiquinone) to oxygen directly, forming superoxide anion. (Paradoxically, a similar phenomenon occurs with hypoxia.) This formation of superoxide anion can be blocked by dissipating the hydrogen ion gradient (e.g., by overexpression of uncoupling proteins) or by preventing transfer of electrons into the transport chain. In larger arteries, uncontrolled endothelial uptake and oxidation of free fatty acids, which are excessively abundant in type 2 diabetes, further exacerbate the energy surfeit and excess generation of superoxide anion.
Although superoxide anion does not penetrate membranes, a substantial amount of the excess superoxide is formed on the outer side of the mitochondrial inner membrane and can be transferred to the cytosol through the voltage-dependent anion channel in the outer mitochondrial membrane. A modest or transient increase in superoxide production might be expected to produce primarily a transient rise in hydrogen peroxide and potentially stimulate an antioxidant response through Nrf2 signaling, as apparently occurs with exercise. Such a response is seen with short-term incubation of endothelial cells in high glucose. However, prolonged incubation, as in diabetes or with frequently repeated although transient exposures to high glucose, appears to result in sufficient superoxide production to overwhelm SOD antioxidant defenses and results in nuclear (as well as mitochondrial) DNA damage, such as single-strand breaks. In response, a DNA repair enzyme, poly(ADP-ribose) polymerase 1 (PARP-1), is activated. PARP-1 uses NAD + as a substrate and adds long ADP-ribose chains to various proteins and transcription factors, to itself, and, importantly, to histones. ADP-ribosylation of histones alters their conformation and allows greater access to the damaged DNA for other repair enzymes. Hydrogen peroxide, although less reactive than other ROS, can also activate PARP-1 (perhaps through formation of highly reactive hydroxyl radical), but to a lesser extent than superoxide and peroxynitrite. PARP-1 can signal for apoptosis or extensive inflammation in the setting of extensive DNA repair, and pharmacologic inhibition or genetic deletion of PARP-1 decreased inflammation and reduced atherosclerosis in hyperlipidemic mice. Activated PARP-1 leads to inhibition of glyceraldehyde-3-phosphate dehydrogenase with accumulation of the 3-carbon substrates, with subsequent promotion of AGE synthesis as well as diacylglycerol production with stimulation of PKC.
Stimulation of the expression of Nrf2 (by inactivation of Keap1 with sulforaphane, a naturally occurring substance in broccoli) upregulated antioxidant defenses and led to a marked reduction in the adverse effects of hyperglycemia, further supporting the role of ROS in mediating endothelial dysfunction in diabetes. Lipoic acid is an important mitochondrial antioxidant, and supplementation was shown to decrease atherosclerosis in hyperlipidemic, diabetic mice. Heterozygous deficiency of lipoic acid synthase in such mice resulted in a 48% increase in atherosclerosis extent.
There is growing evidence for selective endothelial insulin resistance for the vasodilating Akt pathway while the vasoconstricting ERK1/2 pathway (through Shc, Grb2-Sos, Ras, Raf, and MEK1/2) is left intact. The vasoconstriction seems to be particularly promoted through the activation of PKC by fatty acids, such as palmitate. PKC both inhibits insulin signaling through IRS-1 and Akt and promotes signaling through the ERK1/2 pathway. Perhaps more relevant are increases in adhesion molecule expression in endothelial cells after exposure to insulin. Insulin-induced expression of VCAM-1 in endothelial cells was recently shown to be mediated through the insulin-like growth factor 1 receptor and could be completely abrogated by inhibiting the MAPK p38. Interestingly, insulin also stimulated expression of ICAM-1 by way of the insulin receptor. In both cases, MEK1 appeared to be involved but primarily by activating p38 rather than ERK1/2. Endothelial dysfunction (including impaired eNOS phosphorylation and production of NO) was clearly induced recently by exposure to saturated free fatty acid (particularly palmitate) regardless of insulin signaling (with use of mice lacking insulin receptors in all the vasculature or knockout of Akt). These mice also developed hypertension.
Autoimmune and Inflammatory Disease
Increased risk of atherosclerotic disease has been documented for several autoimmune diseases, including systemic lupus erythematosus, rheumatoid arthritis or elevated rheumatoid factor, systemic sclerosis, and psoriasis. It would be reasonable to expect that the elevated plasma cytokines, including TNF-α and IL-6, seen particularly in systemic lupus erythematosus, would activate endothelial cells and thereby initiate atherosclerotic processes, particularly at atherosclerosis-prone sites. Other mechanisms have recently been forwarded. Mice that lack functional Fas ligand or Fas have impaired ability to clear apoptotic debris and have features of autoimmune disease. When crossed with apo E–deficient mice, these mice have increased atherosclerosis. Apoptotic debris may present autoantigens to dendritic cells and thereby lead to autoantibody production and increased circulation of immune complexes, which could cause immune injury and promote inflammation. Alternatively, apoptotic debris itself could directly promote inflammation in atherosclerotic plaques.
Several older studies illustrated a potential synergism between immune injury and hyperlipidemia. Rabbits made only mildly hypercholesterolemic (200 to 250 mg/dL) and subjected to repeated injection of horse serum (a form of immunologic assault with antigen-antibody deposition on lesion-prone areas of endothelium) developed atherosclerosis that was more similar to human atherosclerosis than the usual severe hypercholesterolemia-induced lesions in rabbits. The rabbits appeared to be protected by antihistamines in this setting. A counterpart in humans was suggested to be the finding of increased levels of serum antibodies to heat-dried cow’s milk and boiled egg in patients with coronary heart disease. The display of HSP-60 on endothelial cells in association with activation of endothelial cells from a variety of initiating factors could lead to autoimmune reactions, endothelial damage, and increased atherosclerosis, especially coupled with hyperlipidemia and other risk factors.
A large literature has accumulated relating to immune activation by repeated or latent infections, which suggests another potential mechanism for endothelial activation and atherosclerosis initiation. Macrophage activation may be involved as well. Infection of apo E–deficient mice with Chlamydia pneumoniae or herpesvirus accelerated atherosclerosis. Indeed, so did nonspecific activation of TLR2 receptors. However, treatment of coronary patients with macrolide antibiotics failed to alter subsequent event rates. Thus, causality for the infectious disease hypothesis remains to be proved. Furthermore, if the relationship to infectious disease requires only exposure resulting in an immune response, not continued infection, then the hypothesis may not be provable by any antimicrobial intervention.
Cigarette Smoking
Smoking has long been considered an endothelial cell stressor and activator. A genome-wide analysis of expression changes in 35,000 genes was recently reported after exposure of cultured endothelial cells to cigarette smoke extract. There was massive upregulation of genes related to the unfolded protein response, thought to be due to immense oxidative stress. Generation of free radicals was apparently mediated by metals as well as by reactive species in the extract. This was accompanied by mitochondrial dysfunction, upregulation of HSF1 and heat shock proteins (including HSP-60), and cell cycle arrest. These observations are consistent with prior reports of the pro-oxidant effects of smoking leading to activation of endothelial cells. The rapid reversibility of cardiovascular risk after smoking cessation suggests that this activation affects not only early endothelial activation but precipitating factors such as clotting and stability of advanced plaques as well (probably also through inflammatory effects). Nevertheless, some evidence suggests that persistent inflammatory effects can last for years after smoking cessation.
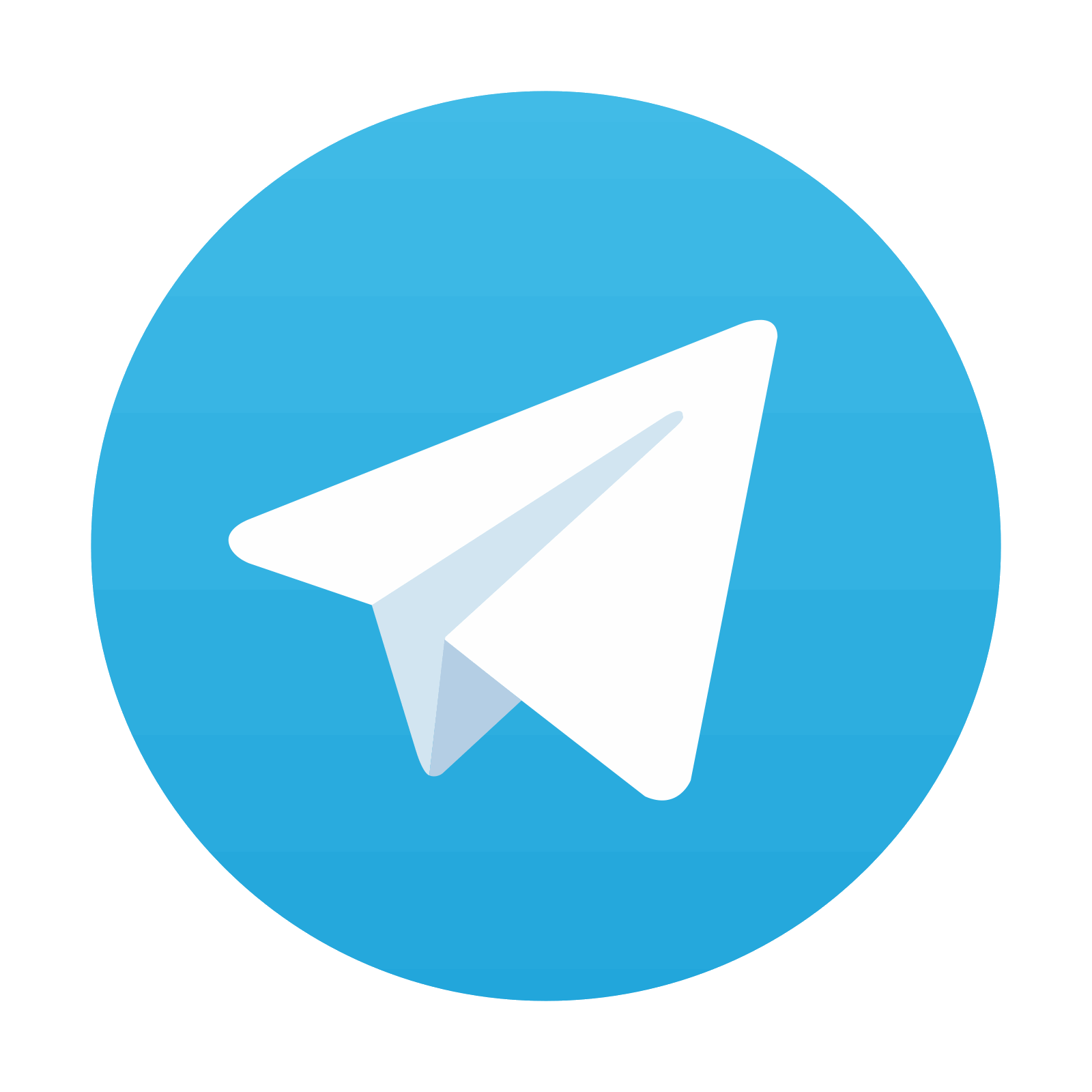
Stay updated, free articles. Join our Telegram channel
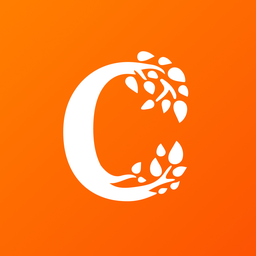
Full access? Get Clinical Tree
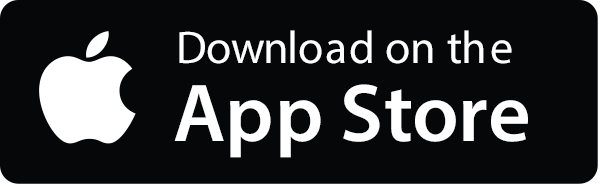
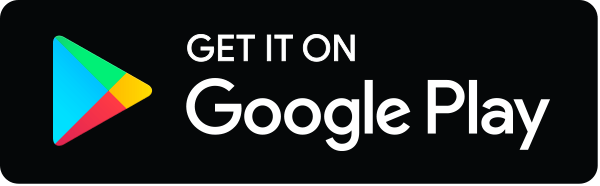