Key Points
- •
Advances in vascular biology and imaging technology have greatly contributed to further elucidation of the complexities of vascular structure and function and the significance of the endothelium in the development of atherosclerosis.
- •
Vascular endothelium is a vast dynamic paracrine system that regulates several key biologic and molecular functions serving to maintain vascular health and homeostasis.
- •
The endothelium functions primarily to modulate vascular tone/vasomotion, to maintain an anticoagulant/profibrinolytic state, to inhibit platelet aggregation and adhesion, to inhibit vascular smooth muscle cell proliferation and migration, and to maintain an anti-inflammatory milieu.
- •
Endothelial activation refers to the biologic response to impairment in vascular homeostasis that engenders a new molecular or functional homeostasis.
- •
Environmental and genetic factors, such as cardiovascular risk factors, impose an oxidative stress on the vasculature through mechanisms such as the nitric oxide pathway.
- •
Translational research from experimental discoveries in vascular biology to the clinical arena has spawned applications of new and emerging invasive and noninvasive techniques to measure endothelial function and dysfunction.
- •
Ongoing research in the development and application of primarily noninvasive imaging techniques to measure endothelial function and dysfunction continues in the pursuit of tests for subclinical disease states, targeting therapeutic strategies and prognosis.
Cardiovascular disease incurs a major burden to the public health and health care system. In the past several decades, advances in vascular biology have greatly contributed to our understanding of the complexities of vascular structure and function and the significance of the endothelium in the development of atherosclerosis. Atherosclerosis is a ubiquitous, complex disease process that is dynamic and multifactorial. Genetic and various environmental factors, with their complex interactions, lead to the initiation and progression of atherosclerosis during decades. The vascular endothelium, the largest paracrine organ, vastly forms the inner lining of all blood vessels in the vasculature to maintain vascular homeostasis through myriad complex biologic properties and physiologic processes. Strategically located between the vessel lumen and smooth muscle layer, the endothelium serves to modulate vascular tone, cell growth, platelet and leukocyte interactions, inflammation, and thrombogenicity.
Physical and chemical stimuli in the vascular milieu initiate and propagate diverse physiologic and molecular processes through signal transduction mechanisms not yet fully understood and through the elaboration and secretion of various substances. The magnitude and duration of exposure to cardiovascular risk factors impose injury on the vasculature, primarily through oxidative stress mechanisms that promote procoagulation, inflammation, vasoconstriction, and proliferation of cell growth. The complexity of atherosclerosis translates into a continuum of cardiovascular disease processes that manifest derangements in endothelial functions, which begin early in the pathogenesis of disease. The study of endothelium from cell to organ system poses the challenge for development and application of various genetic and chemical biomarkers and imaging modalities for diagnosis, therapeutics, and prognosis.
Various invasive and noninvasive imaging modalities have evolved in the last several decades to clinically evaluate structure and function of various vascular beds in health and disease states. Advances in experimental and clinical research underscore the complexity of vascular homeostasis. Perturbations in vascular homeostasis induced by environmental factors or the local biologic milieu are manifestations of endothelial dysfunction that require further definition of the specific vascular property involved. The term endothelial activation refers to the biologic response to impairment in vascular homeostasis that engenders a new molecular functional homeostasis.
Imaging techniques to assess endothelial function, particularly vascular vasomotion, emerged concurrently with the advances in vascular biology, coupled with a keen interest in the use of biomarkers and imaging modalities and techniques to detect subclinical disease while targeting preventive and therapeutic interventions. Investigation continues in the clinical applications to enhance early detection and to guide therapy and prognosis.
Endothelium and Endothelial Physiology: General Overview
Malpighi’s discovery in the 17th century of the endothelium as a physical separation between blood and tissue with no substantial functionality persisted through the 19th and mid-20th centuries. The endothelium is a 0.2- to 4-µm-thick monolayer of squamous endothelial cells lining the entire surface of the vasculature, including endocardium, arteries, arterioles, capillaries, venules, veins, adventitial vasa vasorum, and other microcirculation. Once thought to be a passive, semipermeable membrane between blood flow and vascular wall, the endothelium is essentially a vast autocrine, paracrine, and endocrine organ that spans a surface area of approximately 700 m 2 .
The endothelium is strategically located at the interface of the blood circulation, blood components, and vascular smooth muscle and adventitia. As such, the endothelium is the major regulator of vascular homeostasis, which occurs through myriad diverse and interrelated physiologic functions. These include the regulation of vasomotion, smooth muscle cell proliferation, inflammation, thrombolysis, homeostasis, platelet aggregation, immune responses, cell proliferation, and free radical production. Normal healthy endothelium exerts a variety of effects to maintain vascular homeostasis of these various biologic functions ( Table 32-1 ). It performs these functions through an elaborate array of secretable substances and signal transduction mechanisms in response to a number of different physiologic, chemical, and mechanical stimuli within and around its surrounding milieu and external environmental factors.
|
Healthy endothelium |
|
One of the pivotal roles of the endothelium is the modulation of vascular tone, caliber, and blood flow in response to neural, humoral, and mechanical stimuli by synthesis and release of various vasoactive substances. Specifically, the endothelium plays a key role in regulation of hemostasis and thrombosis, vascular tone, inflammation, and vascular growth and remodeling under normal conditions. Laminar stress is among the most important stimuli that help maintain the normal physiologic state of the endothelium. This is attained by reduction of biologic activity of proteins by S -nitrosylation of cysteine residues and oxidative phosphorylation in mitochondria. However, during exposure to risk factors such as hypertension, diabetes mellitus, and tobacco smoking, dysfunction of endothelium ensues, thus disturbing the fine balance and subsequently resulting in atherosclerosis.
Vasomotion
Regulation of vascular tone by endothelium is achieved by generation and secretion of vasoactive substances. The endothelium exerts a significant effect on both vasodilation and vasoconstriction. Endothelium-mediated vasodilation is predominantly achieved by nitric oxide (NO) and prostacyclins (PGI 2 ). Endothelium-mediated vasoconstriction is regulated by secretion of angiotensin II, platelet-derived growth factors, platelet-activating factor, and endothelin 1, all of which have vasoconstrictive effects. NO serves as an important vasodilator and forms a major basis for endothelial function and dysfunction.
Prostacyclin, another vasodilator synthesized in the endothelium, is a product of arachidonic acid, which is released from membrane phospholipids in response to shear stress. Vasodilator effects of prostacyclins are dependent on expression of receptors in vascular smooth muscles. This limits the role of endothelium-mediated vasodilation in vascular beds where receptors are not expressed. Prostacyclins do not contribute to the maintenance of basal vascular tone of large conduit arteries. Prostacyclins mediate their effect through receptors coupled to adenylate cyclase and elevation of cyclic adenosine monophosphate (cAMP) levels in vascular smooth muscles, stimulation of ATP-sensitive potassium channels, hyperpolarization of cell membrane, and thus inhibition of the development of contraction. Inhibition of the contractile mechanism is also mediated by the expelling of calcium from the cytosol of vascular smooth muscles. In addition, prostacyclins contribute to release of NO by endothelial cells and have a synergistic effect with NO on antiplatelet activity.
Endothelin 1
Endothelin 1 (ET-1) is a potent vasoconstrictor generated in the endothelial cells along with other cell lines. It belongs to a family of structurally related peptides including ET-1, ET-2, and ET-3. Mature human ET-1 is derived from a precursor, preproendothelin 1, through an intermediate molecule and requires a peptidase named ET-converting enzyme. This peptidase serves as a critical physiologic regulator of ET-1 activity. Shear stress and cyclic stress, along with other factors such as hypoxia, stimulate generation of ET-1 from endothelial cells. The vasoconstrictor effect of ET-1 is mediated by binding to ET A on vascular smooth muscle cells. The effect on NO and PGI 2 generation is mediated by binding to ET B receptors on the endothelium.
Vascular beds exhibit heterogeneity in vasoconstrictor response to ET-1. Whereas the renal endothelium and the coronary endothelium are extremely sensitive to ET-1, the pulmonary circulation exhibits a less sensitive response. In addition to vasoconstriction, ET-1 exhibits a wide range of activities. It has a positive inotropic effect on cardiomyocytes and stimulates release of atrial natriuretic peptide from atrial myocytes. In addition, it aids in release of aldosterone and catecholamines from the adrenal cortex and medulla. ET-1 augments the vascular actions of other vasoactive peptides (such as angiotensin II, norepinephrine, and serotonin), participates in leukocyte and platelet activation, and thus facilitates a prothrombotic state. It also has an inhibitory effect on renin release from juxtaglomerular cells. Finally, it enhances release of endothelium-derived relaxing factor and PGI 2 and modulates vascular remodeling.
Inflammation
The endothelium, as a result of its location, serves as a potent anti-inflammatory tissue. It is constantly exposed to various pathogens, anti-inflammatory cells, and immunoreactive substances. Disease states or exposures such as hypertension and atherosclerosis constitute inflammatory changes in the vascular wall. Inflammatory changes include altered expression of adhesion molecules. Under shear stress, endothelial cells exhibit a number of anti-inflammatory properties. This response includes prevention of adhesion of circulating inflammatory cells to endothelial cells and release of immunoreactive substances. NO released from endothelial cells aids in limiting leukocyte adhesion. Furthermore, NO has an inhibitory effect on release of prothrombotic substances, such as von Willebrand factor and P-selection.
NO limits activation of nuclear factor-κB (NF-κB) and thus inhibits adhesion molecule expression and subsequent attachment of immune cells to endothelium. Endothelial dysfunction, as a result of oxidative stress in the endothelial cells, results in activation of NF-κB, a redox-sensitive transcription factor. Subsequent steps include release of chemoattractant proteins such as monocyte chemotactic protein 1 and expression of adhesion molecules (E-selectin, P-selectin, intercellular adhesion molecule 1 [ICAM-1], and vascular cell adhesion molecule 1 [VCAM-1]). This results in monocyte attachment and rolling through the selectins, activation through selectins and chemokines (CCL2, CXCL8, and platelet-activating factor), arrest and adherence through the immunoglobulin G family (ICAM-1, VCAM-1) and integrins (α V β 3 ), and finally extravasation through platelet/endothelial cell adhesion molecule 1. Other molecules involved in extravasation of leukocytes include cadherins.
Hemostasis and Thrombosis
The endothelium serves as a lining of a compartment that maintains blood flow while permitting delivery of important nutrients to other organs in the body. It serves this purpose by inhibiting platelet aggregation and clotting cascade activation. It achieves the antithrombotic properties by generating anticoagulants (antithrombin III, thrombomodulin, tissue factor pathway inhibitor, protein C, and heparan sulfate proteoglycans), fibrinolytics (tissue-type plasminogen activators and urokinase-type plasminogen activators), and platelet inhibitors (NO, prostacyclins, and ADPase [CD39]). On the opposite end of the spectrum, the endothelium maintains hemostasis by production and release of procoagulants (thrombin receptor, protein C receptor, tissue factor, and coagulation factor binding sites) and antifibrinolytics (plasminogen activator inhibitor 1) and by promotion of platelet activation (von Willebrand factor and platelet-activating factor).
Whereas normal endothelial cells do not express procoagulants such as tissue factor, activated endothelial cells rapidly express the same on their cell surface. Similarly, von Willebrand factor is stored in endothelial cells in granules called Weibel-Palade bodies, which are exposed on the endothelial surface in response to injury and other soluble mediators, resulting in formation of a hemostatic plug and platelet adhesion.
Vascular Growth and Remodeling
With better understanding of developmental biology, it is now evident that a close relationship exists between endothelial cells and the hematopoietic cell lineage. It is now known that hemangioblasts may be the common precursor for both cell lineages. The hemangioblasts differentiate into either blood cell precursors or angioblasts, which are endothelial cell precursors. Vessel growth or angiogenesis after the initial process of development of blood vessels involves formation of capillary sprouts by endothelial cells. This process subsequently leads to remodeling and formation of a mature vessel.
Endothelial cells play an important role in the remodeling process during development by secretion of substances that recruit undifferentiated mesenchymal cells and cause their maturation into pericytes or smooth cells. During endothelial dysfunction, in adult cells, vascular smooth muscle proliferation takes place in the presence of oxidative stress and as a result of decrease in endothelium-derived NO, which has an inhibitor effect on vascular smooth muscle growth.
It is now known that the endothelium along with the vasa vasorum contributes to angiogenesis and neovessel formation in atheromas. These neovessels are instrumental in atherosclerotic plaque progression, instability, and rupture. The microvascular channels formed by invagination of endothelial lumen may also serve for the transport of inflammatory cells, such as leukocytes. This is supported by selectively increased VCAM expression on microvascular endothelial cells. In addition to leukocyte migration, microvessels cause intraplaque neovascularization, thus leading to hyperpermeability, and result in microhemorrhage and thrombosis. Evidence of hemosiderin deposits in the neovascular plexus and their colocalization with thrombotic factors such as von Willebrand factor suggest hemorrhage and thrombosis within the atheroma. Hyperpermeable neovessels allow extravasation of red blood cells. Lysis of red blood cells contributes to plaque progression by lipid expansion as their membranes are rich in cholesterol and generation of reactive oxygen species and macrophage activation. As discussed elsewhere, reactive oxygen species deplete endothelium-derived NO and thus limit its inhibitory effect on vascular smooth muscle growth.
Nitric Oxide Pathway
In the early 1980s, Furchgott and Zawadski first demonstrated the obligatory role of the endothelium for vascular relaxation in response to vasoactive substances such as acetylcholine and postulated the existence of an endothelium-derived relaxing factor, later discovered to be NO. Of all substances secreted or controlled by the endothelium, NO is the most recognized molecule; it is pivotal in maintaining vascular tone and mediates inhibition of coagulation, platelet activation, smooth muscle cell proliferation, and inflammation. Inadequate or lack of NO is implicated in endothelial dysfunction.
NO is produced by the endothelial cells from l -arginine ( Fig. 32-1 ). It is a heterodiatomic lipophilic free radical that is synthesized by endothelial nitric oxide synthase, a heme-containing NAD(P)H-dependent oxygenase that requires cofactors tetrahydrobiopterin and nicotinamide adenine dinucleotide phosphate. Three distinct isoforms of nitric oxide synthase (NOS) are known to exist: endothelial NOS (eNOS), inducible NOS (iNOS), and neuronal NOS (nNOS). All three isoforms belong to a family of arginine hydroxylases. Most of the NO production takes place in invaginations of the cell membrane (caveolae) of endothelial cells. It is made from the amino acid l -arginine, which is converted to l -citrulline by means of eNOS. Caveolin 1 regulates the activity of eNOS by binding to calmodulin, with the resultant inhibition of eNOS. However, eNOS activation and NO production are achieved by binding of calcium to calmodulin, causing a displacement of caveolin.

Activity and synthesis of eNOS are modulated by physiologic inhibitors, such as asymmetric dimethylarginine and geranylgeranyl pyrophosphate (intermediate of cholesterol biosynthesis), or pharmacologic inhibitors, such as N G -monomethyl- l -arginine ( l -NMMA) and l -nitroarginine methyl ester. NO, a potent endogenous vasodilator, is basally secreted in response to various physiologic agonists, physical stimuli, and pharmacologic agents. It exerts its effect on vascular tone in multiple ways. The basal secretion of NO maintains the vessel in a vasodilatory state and assists in maintaining vascular health through its various antiatherogenic properties.
Once it is secreted, NO diffuses into the subendothelium and exerts a vasodilator effect on vascular smooth muscle cells. It activates the soluble guanylate cyclase, followed by an increase of the intracellular concentration of cyclic guanosine monophosphate. This results in a reduction in the intracellular calcium concentration, followed by a relaxation of vascular smooth muscle cells. In addition, it decreases the expression and activity of the potent vasoconstrictor ET-1. NO has an inhibitory effect on vascular smooth muscle proliferation and migration and extracellular matrix production. NO exerts this effect by inhibiting the activation of NF-κB. This particular attribute contributes to anti-inflammatory as well as to antiproliferative properties. NO exerts its inhibitory effect on platelet activation and aggregation by stimulation of the cAMP pathway. Its effect on the fibrinolysis system is mediated by stimulation of tissue plasminogen activator release. However, the effect on mobilization of progenitor cells and stem cell modulation of their survival and function are areas of immense interest.
Diminished NO activity may be due to four distinct causes: decreased expression of eNOS enzyme, eNOS uncoupling, increased scavenging of NO, and impaired transmission of NO-mediated signaling. Both physical and humoral stimuli can activate the transcription of eNOS gene. Activation of eNOS by physical stimuli such as shear stress is mediated by Raf, Ras, and ERK1/2 and NF-κB binding to shear stress response element. Humoral factors influencing eNOS transcription include growth factors (vascular endothelial growth factor, basic fibroblast growth factor, epidermal growth factor, and transforming growth factor-β), cytokines, and oxygen radicals.
Uncoupling of eNOS refers to the process by which eNOS switches its predominant function of NO generation to reactive oxygen species formation. When this switch occurs as a result of tetrahydrobiopterin deficiency, generation of oxidants such as superoxide is dominant. However, deficiency of l -arginine also leads to production of hydrogen peroxide. Superoxide anions rapidly interact with NO to form peroxynitrite. Peroxynitrite in turn decreases tetrahydrobiopterin and results in increased consumption and reduced production of NO. Figure 32-2 summarizes the mechanism of decreased NO bioavailability mediated by reactive oxygen species. In addition, various factors known to regulate eNOS gene expression mediate endothelial function and dysfunction ( Table 32-2 ).

Factors | Effect |
---|---|
Physical factors | |
Shear stress | Stimulation |
Hydrostatic pressure | Stimulation |
Humoral factors | |
Reactive oxygen species | |
Nitric oxide | Inhibition |
Oxidized low-density lipoprotein | Inhibition |
H 2 O 2 | Stimulation |
Inflammatory factors | |
Tumor necrosis factor-α | Inhibition |
Growth factors | |
Vascular endothelial growth factor | Stimulation |
Basic fibroblast growth factor | Stimulation |
Epidermal growth factor | Stimulation |
Transforming growth factor-β | Stimulation |
Peptide hormones | |
Angiotensin II | Stimulation |
Endothelin 1 | Stimulation |
Erythropoietin | Stimulation or inhibition |
Endothelial Dysfunction
No single definition of endothelial dysfunction exists, given the complex and ubiquitous nature of endothelial biology. Endothelial dysfunction was first described as structural changes or loss of anatomic integrity in the context of atherosclerosis. Endothelial dysfunction includes broad regulatory changes leading to abnormal vasomotion and the expression of a prothrombotic and proinflammatory phenotype of the vascular endothelium. This poses a challenge to define endothelial dysfunction in terms that include all the possible alterations in vascular homeostasis and at various time points in various disease states.
Endothelial dysfunction encompasses any alterations in the diverse vascular biology and function, particularly vasomotor function as well as the prothrombotic, proinflammatory, proatherogenic properties. Endothelial activation refers to the biologic response to alterations or impairment in vascular homeostasis that would elicit new molecular or functional homeostasis, including gene activation and the repair response to damaged endothelium.
Chronic exposure to injury stimuli and reactive oxygen species may surpass the inherent capacity of the endothelium to mount a suitable defense. This may subsequently lead to aging and senescence of the endothelial cells. Finally, the senescent endothelial cells may detach and be released in the circulation.
Activated endothelial cells, apoptotic cells, and their components in the circulation thus may serve as markers for endothelial dysfunction. The levels of these cell types and their components have been shown to be increased in several inflammatory states, such as coronary artery disease, rheumatoid arthritis, and systemic lupus erythematosus. Endothelial repair may be due to repopulation of the denuded endothelium by replication of adjacent mature endothelial cells or from circulating endothelial progenitor cells.
Endothelial progenitor cells are circulating precursors recruited from the bone marrow, partly by NO-dependent mechanisms. These cells may transform into mature endothelial cells and contribute to maintenance of endothelial integrity and health. The extent to which this mechanism may be successful depends on the exposure to cardiovascular risk factors and inflammatory stimuli. Under certain circumstances, these cells may transform into macrophages and thus may contribute to endothelial dysfunction. Modification of risk factors by interventions such as exercise and statins, as discussed later, may help in mobilization of endothelial progenitor cells and aid in repair. Any imbalance between injury and repair may result in endothelial dysfunction. Whereas levels of endothelial progenitor cells may be increased on exposure to acute insult, chronic inflammatory states result in reduced levels. Reduced levels of endothelial progenitor cells may be a result of overconsumption, inhibited mobilization, or accelerated senescence. Risk factors, by modulating the levels of oxidative stress or NO activity, may influence the levels of circulating progenitor cells. The precise underlying mechanism needs to be understood better.
Oxidative Stress and Endothelial Dysfunction
Oxidative stress refers to a state whereby the rate of formation of reactive oxygen species exceeds the capacity of physiologic antioxidant defense mechanisms. Chronic exposure to reactive oxidative species may overwhelm the inherent antioxidative mechanism and thus contribute to endothelial dysfunction or prolonged endothelial activation. Several enzyme systems are sources of free radical production (reactive oxygen species), including NAD(P)H oxidases, nitric oxide synthases, lipoxygenases, cyclooxygenases, oxidoreductases, and mitochondrial oxidases. Balance between production of reactive oxygen species and activity of superoxide dismutase during oxidative phosphorylation is maintained in normal physiologic conditions.
An abundance of reactive oxidant species alters several important physiologic functions, including regulation of blood flow, vasodilation, coagulation, inflammation, and cellular growth, and activates multiple signaling pathways in vascular wall cells that also contribute to the impairments of vascular function and repair. On exposure to risk factors such as obesity and diabetes mellitus, there is an alteration in this balance due to increased substrate delivery, such as circulating free fatty acids. In coronary artery disease, increased oxidative stress is a result of increased nicotinamide adenine dinucleotide phosphate oxidases and xanthine oxidase. As depicted in Figure 32-2 , diverse risk factors result in increased oxidative stress and contribute to decreased NO bioavailability by three distinct mechanisms. Production of superoxide is increased in the diseased vessels of patients with coronary vascular disease. Superoxide interacts with NO, forming peroxynitrite anions, resulting in consumption of NO and loss of its activity.
NO is a key mediator in vascular homeostasis as it serves as an antiatherogenic molecule, promotes vasodilation, and counteracts inflammation, platelet aggregation, and vascular smooth muscle proliferation. A large body of evidence supports the tenet that endothelial dysfunction is caused to some degree by accelerated inactivation of NO by reactive oxygen species, although exact mechanisms are not entirely elucidated and strategies for targeted therapy are still under investigation. Another mechanism is the redox-dependent inhibition of the enzyme dimethylarginine dimethylaminohydrolase. This results in increased concentration of the endogenous eNOS inhibitor asymmetric dimethylarginine (ADMA). Levels of ADMA are elevated in a large number of conditions, including several associated with risk of endothelial dysfunction. ADMA concentration may be increased because of impaired excretion or increased synthesis, as in the case of vascular shear stress. Accumulation of ADMA acts as a competitive antagonist to the eNOS substrate l -arginine.
Role of Endothelial Dysfunction in the Presence of Specific Risk Factors and Atherosclerotic Disease
Several risk factors may play a critical role in contributing to endothelial dysfunction and thus atherosclerosis. Traditional and novel cardiovascular risk factors, including smoking, aging, hyperlipidemia, hypertension, diabetes, and family history of premature atherosclerosis, among others, are associated with a loss or attenuation of endothelium-dependent vasodilation in both children and adults. Elevated C-reactive protein, chronic systemic infection, obesity, and several immune-mediated diseases are also associated with impaired endothelial function. Given its complex biology, the term endothelial dysfunction applies broadly to the various perturbations that contribute over time to the development and clinical expression of atherosclerosis.
The underlying mechanism through which risk factors impart vascular injury initiating endothelial dysfunction is indeed multifactorial, yet it is generally accepted that the predominant mechanism is oxidative stress and redox injury resulting in decreased synthesis or increased degradation of NO. Given the diverse vasculoprotective properties of NO and related pathways, there is increasing evidence to support the notion that endothelial dysfunction begins with impairment of oxidative stress, with subsequent unavailability of NO. Factors that help stimulate the production and release of NO have also been an area of active research.
An important mechanical stimulus that evokes vasodilator release of vasoactive substances, particularly NO, from the vascular endothelium is pulsatile flow and shear stress induced from movement of blood along the endothelial cells. Varying degrees of shear stimulus have been shown to have an impact on the degree of vasodilation, which points to the complexity of the underlying vasomotor function, the impact of vascular risk factors and disease states, and the ability to decipher specific mechanisms underlying impaired endothelial vasomotor function. Reactive hyperemia is a transient increase in blood flow, physiologically or mechanically invoked, that immediately follows a designated period of vessel occlusion or flow disturbance. High shear stress on the endothelium provides the stimulus for NO release. In addition, a variety of vasodilators, such as adenosine and hydrogen ions, among others, are released and act locally on the microvessel milieu.
Endothelial Vasomotor Function Testing
The initial observations and discoveries in vascular biology since the 1980s stimulated intense basic research in vascular biology in the ensuing decades to further delineate the various components and mechanisms of vascular diseases. A vast body of work emerged emphasizing the importance of endothelium-derived NO as a potent endogenous vasodilator that contributes to vascular health in optimal conditions. Moreover, these observations formed the basis for translational research to study the characteristics and clinical significance of endothelium-dependent properties, such as vasodilation, in various vascular beds and in various vascular disease states.
Experimental and clinical techniques using physiologic agents and various imaging techniques were developed and applied to study vascular structure and functions of both conduit and resistance vessels in the normal state and within the context of cardiovascular risk factors, such as dyslipidemia, hypertension, diabetes, and early atherosclerosis. Such techniques also spurred further investigations of the impact of therapeutic and lifestyle modifications on endothelial dysfunction and its prognosis.
Invasive Measures of Coronary Vasoactivity
With the discoveries in endothelial biology in the 1980s, there was growing interest in the potential to clinically examine perturbations in vascular function before the advent of detectable atherosclerosis and clinical events. Numerous studies and methods using various physiologic agents and techniques to study endothelium-dependent vasomotor function in conduit and resistance vessels in the normal state and in the presence of cardiovascular risk factors and atherosclerosis ensued. Ludmer and colleagues, in the 1980s, first described impaired endothelium-dependent vasomotor function with intracoronary injection of acetylcholine and quantitative coronary angiography in humans with various degrees of atherosclerosis noted by angiography. They demonstrated that like isolated vascular rings used in basic experimentation, human coronaries that appeared angiographically normal or with mild stenosis paradoxically vasoconstricted to acetylcholine but not to nitroglycerin. Subsequently, endothelium-dependent, NO-mediated vasomotor function testing gained notoriety as a useful tool to assess the functional integrity of vascular endothelium in vivo.
The endothelium-dependent vasodilator response may serve as a surrogate marker for the bioavailability of NO. Endothelial function is most commonly measured as the vasomotor response to pharmacologic stimuli, such as acetylcholine, methacholine, bradykinin, serotonin, papaverine, and substance P, or to a physical stimulus, such as shear stress (increased blood flow velocity), exercise, cold pressor test, and mental stress. During cardiac catheterization, alterations in arterial lumen diameter in response to such stimuli can be measured by computerized edge detection software to provide reproducible measurements of the angiographic lumen diameter compared with baseline diameters. Lumen diameter and coronary flow by quantitative coronary angiography and intracoronary Doppler study, respectively, can be used to assess changes in vessel diameter and coronary flow reserve.
Endothelial function in resistance vessels (microcirculation) is also critical in the assessment of endothelial vasomotor function. Resistance vessels regulate blood flow in response to changes in perfusion pressure (autoregulation) and metabolic needs (metabolic regulation). The vascular tone of resistance vessels determines blood flow; therefore, altered blood flow detected by techniques without considerable changes in mean blood pressure indicates changes in resistance vessel tone. Endothelial function of forearm resistance vessels can be assessed by measurement of coronary blood flow with intravascular Doppler study in response to intracoronary adenosine or by forearm blood flow responses to intra-arterial agonists with strain-gauge plethysmography.
Angiography is invasive and carries some risk, and it is not conducive to repeated studies in the same individual over time or for the study of relatively low risk populations. Insights from these invasive studies of endothelial vasomotor function and flow reserve provoked a keen interest in the pursuit of similar studies in the peripheral circulation with both invasive and noninvasive techniques. This was motivated by the notion that endothelial dysfunction is both a local and systemic physiologic state, along with an interest in detecting the impact of cardiovascular risk factors and the presence of preclinical atherosclerotic disease.
Invasive Assessment of Forearm Microcirculation (Venous Occlusion Plethysmography)
The limitations inherent in coronary artery circulation studies of vasomotion led researchers to pursue the peripheral circulation for further investigation. There was also keen interest to determine whether there were methods to more broadly assess the systemic nature of atherosclerosis and the broader impact of cardiovascular risk factors and the potential role to evaluate the impact of interventions and risk factor modifications. Moreover, further study led to an interest in the relationship of conduit and resistance vessels in vascular health and disease.
Venous occlusion plethysmography is an invasive technique that indirectly measures microvessel function as forearm blood flow in response to an intra-arterial infusion of a vasoactive substance such as acetylcholine, substance P, or adenosine into either the brachial artery or radial artery or to reactive hyperemia (increased shear stress). The standard testing technique is well described, reliable, and highly reproducible and typically used in research protocols. The invasive aspect of this technique with cannulation of peripheral arteries poses the potential for injury to the artery and nerves, which makes it less desirable for routine clinical use and examination of larger populations. It is a valuable research tool to evaluate the pathologic mechanisms underlying endothelial dysfunction and the impact of various therapeutic interventions.
Noninvasive Assessment of Peripheral Conduit Vascular Reactivity: Brachial Artery Ultrasound
Ultrasound techniques have long been used to study vessel physiology. Ultrasound assessment of brachial artery vasoreactivity with high-resolution B-mode ultrasound emerged as a clinical research tool in the early 1990s to noninvasively study endothelium-dependent vasomotor function. In 1992, Celemajer and colleagues used B-mode ultrasound to study the brachial artery’s vasoactive response to increased shear stress induced by hyperemia in adults with coronary artery disease or smoking exposure. The key observation, termed flow-mediated vasodilation (FMD), expressed as a percentage (FMD%), was impaired vasoactivity detectable noninvasively with ultrasound in individuals with risk factors, coronary artery disease, or both. Concurrent with observations from basic studies and invasive coronary studies, flow-mediated vasodilation in the brachial artery is abolished by the NO synthase inhibitor l -NMMA.
Since its inception, flow-mediated vasodilation has been applied widely as a research tool to evaluate the impact of cardiovascular risk factors and preclinical disease states and to improve endothelial function with targeted specific interventions and risk factor modifications. Further exploration of the technique led to studies of underlying mechanisms for the time course after the hyperemic response, the effect of blood pressure cuff occlusion duration, the effect of upper arm occlusion versus lower arm occlusion for technical ease of imaging and targeted NO stimulation, and the effect of other stimuli such as cold pressor and mental stress.
Advances in vascular biology and the role of oxidative stress in enhancing lipid oxidation and promoting a proinflammatory state spearheaded further investigations with this technique. Basic studies of vascular rings exposed to chylomicron remnants and the effect of fat meals with and without antioxidant vitamins on flow-mediated vasodilation contributed to the notion that healthy vascular beds exposed to oxidative stressors manifest acute alterations in endothelial function. Studies that demonstrated significant improvement in flow-mediated brachial artery vasodilation inspired enthusiasm to study the impact of various risk factors and targeted therapies for cardiovascular disease. Correlative studies of brachial artery vasoactivity with intracoronary vasoactive substances and carotid intima-media thickness provided support for brachial artery vasoactivity as an index or marker of endothelial function. Large clinical trials and epidemiologic longitudinal studies now incorporate FMD% as a marker of impaired vasomotor endothelial dysfunction.
Technique
The technique and examination protocol for brachial artery vasoactivity testing are well described in the literature. Seemingly simple, it is technically challenging, with a significant learning curve to achieve high-quality, consistent performance and reproducibility in both technique and interpretation.
Standard ultrasound systems equipped with vascular software with B-mode two-dimensional imaging, color and spectral Doppler display, internal electrocardiographic monitor, and high-frequency vascular linear array transducer (range, 8 to 12 MHz) are required. Higher frequency transducers of 8 to 12 MHz compared with 7 to 7.5 MHz enable visualization of the intima as opposed to the vessel wall alone, which has implications for the application of measurement techniques. Before brachial artery vasoactivity testing, the individual should avoid vasoactive substances or stimuli and rest quietly supine for 10 minutes.
The technical preparation involves positioning of the subject’s arm extended and supinated to allow optimal imaging of the brachial artery antecubitally. Blood pressure occlusion for 5 minutes and release create the shear stress to induce flow-mediated vasodilation.
Application of an upper versus a lower arm cuff for occlusion has been studied, and the placement provides different stimuli. Upper arm occlusion provides a more robust shear stimulus for vasodilation and probably invokes other vasoactive mechanisms besides release of NO. Upper arm occlusion typically elicits an increase in hyperemic flow of four to five times from baseline and greater change in vasodilation compared with lower arm cuff occlusion. Furthermore, vasodilator response to upper arm occlusion hyperemia has been shown to be largely NO mediated. In addition, arteries smaller than 2.5 mm in diameter are difficult to measure, and vasodilation is difficult to perceive in vessels larger than 5.0 mm in diameter.
Flow-mediated vasodilation typically occurs 60 to 90 seconds after cuff release while accurately acquiring the image at the same position compared with baseline. Brachial artery flow returns to baseline by 1 minute after hyperemia, but dilation may ensue beyond 1 minute. The physiologic characteristics of this response may be individually dependent as well as indicative of impaired vascular health in the setting of risk factors or genetic predisposition to disease states such as atherosclerosis. Brachial artery diameter is measured perpendicular to the longitudinal axis in which the lumen-intima interface is visualized on the near (anterior) and far (posterior) walls. The lumen-intima interface or media-adventitia interface is identified with electronic calipers from the ultrasound system analysis software or determined through edge detection computer software typically performed off-line. Edge detection programs that can account for skew by elliptical modeling have less variance in their measurements of brachial artery diameter. Maximal vasodilation after hyperemia may be variable within an individual or in certain disease states, although most individuals dilate maximally at approximately 60 to 90 seconds after cuff release. Consequently, it is recommended that B-mode acquisition of the brachial artery be acquired from 30 seconds up to at least 2 minutes after hyperemia for off-line analysis ( Fig. 32-3 ).

Flow-Mediated Vasodilation
FMD% is expressed as the change in post-stimulus diameter as a percentage of the baseline diameter.
FMD % = post-hyperemic diameter − baseline diameter ÷ baseline diameter × 100
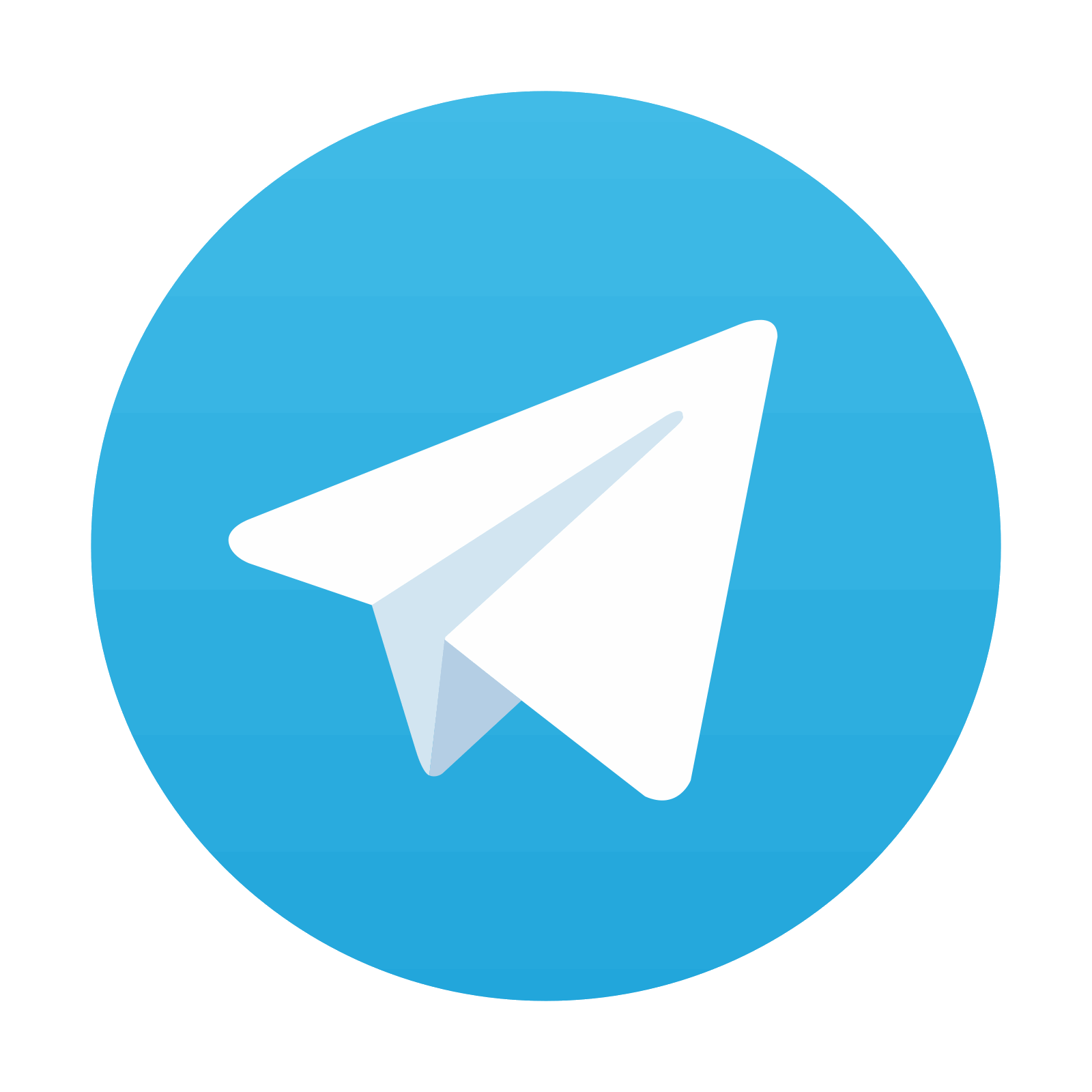
Stay updated, free articles. Join our Telegram channel
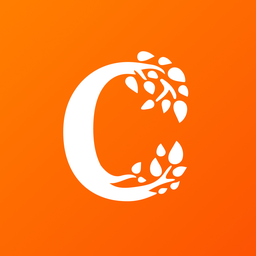
Full access? Get Clinical Tree
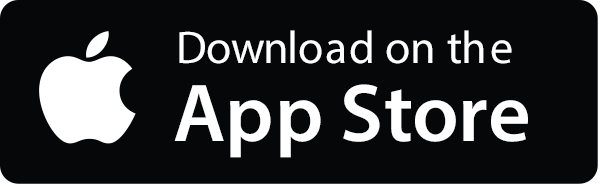
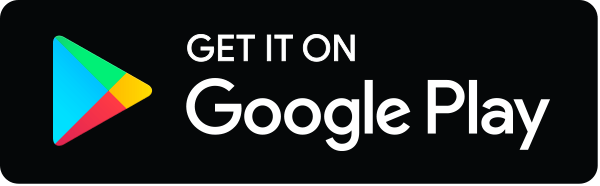