Magnetic Resonance Imaging of the Heart: Introduction
In the last decade, cardiovascular magnetic resonance imaging (CMR) has changed dramatically. Technical and clinical advances have expanded CMR from primarily a tomographic imaging modality, providing static images of morphology, to one that is dynamic, allowing the rapid, high-resolution imaging of ventricular function, valvular motion, and myocardial perfusion. Moreover, CMR is now considered the gold standard for the assessment of regional and global systolic function, myocardial infarction (MI) and viability, and the assessment of congenital heart disease. The aims of this chapter are to provide an introduction to the technical aspects of CMR and to provide an overview of the clinical applications that are available to clinicians today.
Basic Principles
Similar to other medical imaging techniques, magnetic resonance imaging (MRI) acquires images through the transmission and receiving of energy. However, unlike other modalities, MRI offers the capability to modulate both the emitted and received signals so that a multitude of tissue characteristics can be examined and differentiated without the need to change scanner hardware. As a result, from a single imaging session, one could obtain a wealth of information regarding cardiac function and morphology, myocardial perfusion and viability, hemodynamics, large vessel anatomy, and so forth. This information, however, is gathered not from a single long acquisition but rather from multiple short acquisitions, each requiring different pulse sequences (software programs that drive the scanner) with specific operational parameters and optimal settings. Unfortunately, magnetic resonance (MR) vendors may use proprietary names for the same imaging methods and settings.1,2 Thus the goal of this section is to clarify these issues and to provide a simple framework of the technical aspects of MRI. Where appropriate, we will discuss issues specific to CMR.
It is important to recognize that an MRI scanner is not a single device, but rather consists of multiple separate components. A schematic of these components is shown in Fig. 23–1. A detailed explanation of each of these components is beyond the scope of this chapter; however, a basic understanding is useful. For instance, poor image quality may arise from a variety of problems, and the ability to quickly distinguish those that are complex (eg, hardware malfunction that requires servicing) from those that are simple (eg, motion artifact that can be immediately resolved by better communication with the patient) will be highly valuable.
Figure 23–1.
Components of the MRI scanner. For a CMR study, the operator defines the type of examination and manipulates the imaging parameters from a control computer console using a graphical user interface (1). Software, known as a pulse sequence, is selected from a menu to acquire images that are appropriate for the diagnostic question. The precisely timed radiofrequency (RF) pulses, used to stimulate tissues, are generated by the pulse sequence controller (2), RF transmitter (3), and RF coil (4). For CMR, the electrocardiogram signal (5) from the patient is often used for timing. Spatial image information is encoded by the gradient coil amplifier (6) and gradient coil (7), which alter the net magnetic field, known as Bo, of the MRI scanner. The MR signal from the body is detected from fixed receiver coil arrays (8) that are built into the patient table and from flexible arrays that can be placed on top of the patient. These received signals, which are usually analog, are processed and digitized by an analog-digital converter (9) and then passed to a computer dedicated to image reconstruction (10). The final images are then stored in a image database or picture archiving and communication system (11) and can undergo postprocessing at a workstation (12) and interpretation at auxiliary viewing stations (13).
An MRI scanner performs three basic operations (Fig. 23–2): (1) generation of a static magnetic field, (2) transmission of energy within the radiofrequency (RF) range to the patient, and (3) receiving the MR signal after the transmission of RF energy. Most clinical CMR scanners today have a static magnetic field strength (Bo) of 1.5 Tesla, which is 30,000 times stronger than the earth’s magnetic field. This high magnetic field strength is generated by a superconducting magnet that is housed within the MRI scanner itself. When a patient is placed within the bore of the scanner, hydrogen protons within the patient’s body align parallel or anti-parallel to the static magnetic field. More protons align parallel to the field than against the field, leading to a small net magnetization vector (see Fig. 23–2A). The equilibrium distribution of parallel and antiparallel protons is predicted by the classical Boltzmann distribution. While aligned in the magnetic field, these protons rotate or precess about the field (in the same way a spinning top precesses in a gravitational field) at a rate known as the Larmor frequency. This frequency (ωo) depends on magnetic field strength (Bo) and a nuclei-specific physical constant, known as the gyromagnetic ratio (γ), by the formula, ωo= γ Bo. For hydrogen protons precessing in a 1.5-T magnetic field, the Larmor frequency is 63.9 MHz, which is in the radiofrequency range.
Figure 23–2.
Basic operations of the MRI scanner. A. The static magnetic field (Bo). The protons align parallel or antiparallel to the static magnetic field, creating a small net magnetization vector. While aligned to the magnetic field, the protons precess at the Larmor frequency. B. Transmission of radiofrequency energy (RF). Energy is transmitted to the rotating protons by a radiofrequency pulse at the Larmor frequency. RF pulses that result in a flip angle of 90 and 180 degrees are shown (top and bottom, respectively). The figures are presented in the rotating frame of reference, where the x-y axes are rotating at the Larmor frequency and thus appear stationary. C. Generation of the MR signal. Rotation of the net magnetization vector into the transverse plane results in the creation of a time-varying magnetic field, which in turn induces an alternating current in the receiver coil array, which is the MR signal.
The next step toward image generation is the transmission of energy to the region of interest. Importantly, transmission of energy to the precessing hydrogen protons is only possible when the frequency is equal to the Larmor frequency (ie, on resonance). With the absorption of the energy from the RF pulse, the net magnetization vector is tilted from its equilibrium orientation parallel to the static magnetic field (longitudinal direction) into the transverse plane. The angle of displacement of the net magnetization vector is known as the flip angle (see Fig. 23–2B) and may be varied depending on the pulse sequence. Rotation of the precessing net magnetization vector into the transverse plane results in the creation of a time-varying magnetic field. This varying magnetic field induces an alternating current in the receiver coil arrays in the same manner that a spinning electromagnetic generator produces electricity. The signal created from a single RF excitation is illustrated in Fig. 23–2C and is known as a free induction decay.
After the RF excitation, two independent relaxation processes return the net magnetization vector to its thermal equilibrium (realigned with the static magnetic field, Fig. 23–3). The first process, known as longitudinal or spin-lattice relaxation, describes the regrowth of the magnetization vector parallel to the static magnetic field (see Fig. 23–3A). Longitudinal relaxation results from the transfer of energy from the excited protons to surrounding molecules in the local environment. The time constant T1 describes the exponential regrowth of longitudinal magnetization. The second process, known as transverse or spin-spin relaxation, describes the decay of the magnetization vector in the transverse (X–Y) plane (see Fig. 23–3B). Transverse relaxation may take place with or without energy dissipation. For example, the transfer of energy leading to longitudinal relaxation also results in transverse relaxation. Additionally, processes that simply cause proton spins to lose phase coherence without energy dissipation lead to transverse relaxation as well. The latter mechanism most commonly results from static or slowly fluctuating variations in the magnetic field within the imaged sample. The time constant T2 describes the exponential decay of transverse magnetization. The T1 and T2 are intrinsic properties of any given tissue. Pulse sequences use differences in T1 and T2 to generate image contrast between tissues.
Figure 23–3.
Longitudinal recovery and transverse decay after a 90-degree flip angle excitation. A. Longitudinal magnetization recovery (T1 relaxation). The green growth curve demonstrates the exponential regrowth of the longitudinal component of the net magnetization vector. B. Transverse magnetization decay (T2 relaxation). The red decay curve illustrates the exponential decline of the transverse component of the net magnetization vector. C. The net magnetization vector. The vector sum of the longitudinal and transverse components that comprise the net magnetization vector is shown.
The MR signals after RF excitation are localized in three-dimensional space by the use of magnetic fields generated by three sets of gradient coils (Fig. 23–4). These gradient coils alter the strength of the static magnetic field as a linear function of distance from the isocenter of the magnet in each of three orthogonal directions (x-, y-, or z-axes). The variation in field strengths across space produces differences in proton precessional frequencies along each axis. Specifically, to form a two-dimensional image, the gradients allow the selection of the slice of interest (eg, slice encoding direction) and also modulate the MR signals to provide in-plane spatial information along the frequency-encoding direction and the phase-encoding direction. For slice selection, the slice-encoding gradient (z-axis gradient for a transaxial slice) is played during RF excitation (see Fig. 23–4B). Because energy deposition is only possible on-resonance, altering the center frequency of the RF pulse varies the slice location. Increasing or decreasing the bandwidth of frequencies in the transmitted RF pulse increases or decreases the thickness of the imaged slice. For spatial localization in the frequency-encoding direction, the x-axis gradient (for a transaxial slice) is played during MR signal receive (see Fig. 23–4C). As a result, specific frequency components of the MR signal arise directly from specific spatial locations along the x-axis. Spatial localization in the phase-encoding direction is more difficult conceptually. The y-axis gradient (for a transaxial slice) is played for a finite time before MR signal receive. This results in a phase shift in the precessing protons that varies with location along the y-axis. Importantly, each image is the result of multiple MR signal readouts, each of which were preceded by a phase-encoding gradient step, with slight differences in the strength (amplitude) of the y-axis gradient. After all the phase-encoding steps are completed, the raw data from the scanner consists of a two-dimensional grid of data (also known as k-space), which is converted to an MR image by an inverse two-dimensional Fourier transform by the image reconstruction computer (see Fig. 23–1).
Figure 23–4.
Spatial localization of the MR signal. A. Orthogonal magnetic field gradients are used to localize the MR signal. The gradients are shown oriented for a transaxial imaging plane; however, the axes may be oriented orthogonally in any arbitrary direction. B. Slice selection. The RF pulse with center frequency ωo excites proton spins located at position zo along the direction of the gradient when applied in the presence of the linear slice select magnetic field gradient. Changing the RF pulse center frequency to ω1 or ω2 shifts the location of the imaging slice to z1 or z2, respectively. C. Frequency encoding. During MR signal receive, the frequency encoding gradient alters the frequency of the MR signal depending on its position along the direction of the gradient. The MR signal from any given position has a unique frequency. In the example shown, frequency ωL (or ωH) is an MR signal from position XL (or XH).
One of the important advantages of MRI is the ability to generate substantial soft tissue contrast by the use of pulse sequences and the administration of contrast media. In general, pulse sequences are adjusted to emphasize differences in tissue T1 and T2, which may be inherent or altered by the presence of contrast media. For instance, on pulse sequences that are T1 weighted, tissues with short T1, such as fat, appear bright. Traditionally, T1 weighting is accomplished by imaging with short RF repetition times (TR), which magnify differences in longitudinal recovery between tissues. Newer sequences use magnetization preparation pulses, such as saturation or inversion pulses, to create improved T1 contrast (Fig. 23–5). An inversion (180°) compared with saturation (90°) prepulse provides greater T1 weighting, but the sequence is more prone to artifacts when gating is irregular (either because of ECG artifact or arrhythmia). T2-weighted pulse sequences are traditionally created using relatively long echo times (TE). With long TE (on the order of tissue T2), there is increased separation of different T2 relaxation curves, which translates into larger differences in image intensity.
Figure 23–5.
Magnetization preparation pulses to create T1 contrast. A. Saturation recovery. A 90° RF pulse followed by strong gradient spoilers reduces the net magnetization to zero. Magnetization recovers depending on tissue T1. For instance, immediately after intravenous bolus administration of gadolinium contrast, myocardium with normal perfusion has substantial uptake of gadolinium, thus has short T1, and appears bright on saturation recovery perfusion MRI. In comparison, myocardium with reduced perfusion has diminished uptake of gadolinium, longer T1, and appears dark. Ideally, data readout should follow the saturation pulse at a specific time (time point b rather than time point a or c) to achieve maximum separation between the T1 relaxation curves of normal and abnormal myocardium. B. Inversion recovery. An 180° RF pulse inverts the longitudinal magnetization from the +z-axis to the −z-axis. Often used in infarct imaging, the time between the inversion pulse and the center of data readout (inversion time, or IT) is selected to accentuate the differences of gadolinium uptake in normal and infarcted myocardium. Specifically, the inversion time is chosen so that the center of image readout (for linear k-space acquisition) occurs when the T1 relaxation curve of normal myocardium crosses zero (eg, nulled). Note that with this inversion time, the T1 relaxation curve of infarcted myocardium is above the zero crossing and infarcted tissue is bright.
The administration of intravenous contrast agents can also be used to affect image contrast by altering tissue T1 and/or T2. The magnitude of T1 and/or T2 change depends on the specific relaxivities of the contrast media, the distribution characteristics (ie, intravascular, extracellular, or targeted to a specific tissue), and tissue perfusion. Gadolinium-based contrast media are commonly used in CMR. Gadolinium is a lanthanide metal with seven unpaired electrons, making it strongly paramagnetic. When administered, it primarily shortens the T1 in the tissues where it is distributed (Fig. 23–6).
Figure 23–6.
The effect of gadolinium contrast on T1- and T2-weighted imaging. Before contrast administration, there are minimal differences in inherent tissue T1 and T2 between normal and infarcted myocardium, thus infarction is poorly delineated (top panel). After gadolinium administration, the T1 of infarction (although not T2) is markedly shortened, leading to clear delineation on the T1-weighted image (bottom panel). T1-weighted images were acquired using an inversion recovery gradient echo sequence. T2-weighted images were acquired using a dark blood turbo spin echo sequence. Approximate T1 and T2 times are for 1.5-T scanners.
The CMR environment has the potential to pose serious risks to patients and facility staff in several ways. Injuries may result from the static magnetic field (projectile impact injuries), very rapid gradient field switching (induction of electric currents leading to peripheral nerve stimulation), RF energy deposition (heating of the imaged portion of the body), and acoustic noise. The risks of projectile injuries from the static magnetic field are minimized by the institution of policies that strictly limit access to the magnet room. For instance, patients are extensively screened before imaging, and all facility personnel undergo dedicated training in MR safety. The use of MR safe or compatible equipment (stethoscopes, wheelchairs, gurneys, oxygen tanks, infusion pumps, monitors, etc) with clear labeling of such in the scanner area reduces this risk further. The US Food and Drug Administration has placed limits on the rate of change of gradient magnetic fields (eg, the slew rate) and the amount of RF energy (eg, specific absorption rate) that can be transmitted to patients. All scanners monitor the slew rate and calculate the specific absorption rate to help prevent nerve stimulation and heating. Acoustic noise of 100 dB or more is generated from the vibration or motion of the gradient coils during image acquisition. The use of protective hearing devices, such as headphones or earplugs, reduces noise to levels that do not result in hearing impairment or patient discomfort. In practice, continuous communication with the patient throughout the exam is important for patent comfort and safety.
Patients with medical devices or implants may face additional potential hazards, including device heating, movement, or malfunction. For example, ferromagnetic aneurysm clips or electronic medical devices (eg, neural stimulators, insulin pumps) are strict contraindications to MRI. However, there is a specific subset of patients with metallic implants/devices that may safely undergo MRI. A comprehensive list of devices/implants that are compatible with undergoing MRI scanning may be found elsewhere.3,4 Regarding cardiac devices, it is important to note that prosthetic valves and coronary artery stents are now considered safe for MRI scanning.3–5 Indeed, recently, the US Food and Drug Administration approved the use of MRI immediately after the implantation of paclitaxel and sirolimus drug-eluting stents. At most institutions, MRI scans are not performed in patients with implanted pacemakers or defibrillators because of the potential risk of device malfunction, excessive device or lead heating, or induction of currents within the leads. Recently, however, a few preliminary reports have emerged, suggesting that MRI may be possible in patients with modern pacemakers and defibrillators in whom the benefits are deemed greater than the risks.6-10 In patients in whom devices have been extracted, but with the leads remaining (both transvenous or epicardial), MRI is contraindicated because the risk of heating or induction of currents may be higher.
Recently, it has been reported that a small subset of patients with end-stage renal disease receiving gadolinium contrast may be at risk for developing nephrogenic systemic fibrosis.11-14 Nephrogenic systemic fibrosis is characterized by an increased tissue deposition of collagen, often resulting in thickening and tightening of the skin and predominantly involving the distal extremities. Additionally, fibrosis may affect other organs, including skeletal muscles, lungs, pulmonary vasculature, heart, and diaphragm. Thus gadolinium contrast agents should be used cautiously (and alternative tests considered) in patients with severe chronic renal disease (eg, glomerular filtration rates ≤30 mL/min/1.73 m2), particularly those undergoing peritoneal dialysis or hemodialysis. Other at-risk groups include patients with acute renal failure (in whom estimated glomerular filtration rate may not accurately reflect renal function), patients with hepatorenal syndrome, and patients in the peri-transplant period after liver transplant. A policy statement regarding the use of gadolinium contrast agents in the setting of renal disease has been published by the American College of Radiology.11
The Cardiovascular Examination
Although a comprehensive review of pulse sequences is beyond the scope of this chapter, a brief discussion is presented to facilitate understanding of the basic framework of the CMR examination. An individual pulse sequence is a combination of radiofrequency pulses, magnetic gradient field switches, and timed data acquisitions, all applied in a precise order, that results in either accentuation or suppression of specific biological parameters. A simple way to conceptualize pulse sequences is to consider them as consisting of two separate elements: the imaging engine and associated modifiers.15 The imaging engine is a required component that provides information regarding the spatial relationship of objects within the imaging field (ie, it is the main component that produces the image). Modifiers are optional components that can be added to the imaging engine either individually or in combination to provide specific information regarding tissue characteristics or to speed imaging. Figure 23–7 lists some of the more commonly used imaging engines and modifiers in CMR.
Figure 23–7.
MRI pulse sequence structure. The MRI pulse sequence can be considered as composed of two separate elements: the imaging engine and modifiers. Typical images using different imaging engines and modifiers are shown at the bottom. See text for further information. Modified with permission from Shah et al.15
Figure 23–8 depicts the protocol steps, associated pulse sequences, and the timeline of a typical core examination that includes stress testing. Depending on the CMR study indication and the findings during the course of the examination, additional elements may be added to fully investigate the clinical question.
Figure 23–8.
Timeline and potential components of a multi-technique CMR examination for cardiac imaging. Reproduced with permission from Kim et al.195
Scouting is the first and simplest procedure to perform. The goal of scouting is to establish the short- and long-axis views of the heart. Because of patient anatomical variation, both the short- and long-axis cardiac views lie at arbitrary angles with respect to scanner coordinates and are therefore referred to as double oblique planes. In order to obtain these views, a standard procedure should be followed (Fig. 23–9). Scout images are acquired using a single-shot imaging engine (steady-state free precession [SSFP] or half-Fourier acquisition single-shot turbo spin-echo [HASTE]) during free breathing.
Figure 23–9.
Procedure for scouting. In order to establish the short- and long-axis planes of the heart, the following steps are followed. Step 1: Images are obtained along the scanner axes (sagittal, coronal, and axial; 1A,1B, and 1C, respectively). Step 2: From a pseudo four-chamber long-axis view (usually from the axial image), one prescribes a perpendicular plane through the approximate apex, which results in an approximate two-chamber view. Step 3: Another perpendicular plane is prescribed through the apex, which results in a true long-axis view (usually four chamber). Step 4: A perpendicular plane, bread-loafing the heart, delivers the true short-axis plane.
The assessment of cardiac function and volumes is a fundamental component of the core examination. CineMRI, using a gradient recall echo (GRE) or SSFP imaging engine, has been shown to be highly accurate and reproducible in the measurement of ejection fraction, ventricular volumes, and cardiac mass.16 In recent years, cineMRI has become widely accepted as the gold standard for the measurement of these parameters.16 Moreover, it is also increasingly used as an end point in studies of left ventricular remodeling17-19 and as a reference standard for other imaging techniques.20,21Table 23–1 summarizes the literature for these measurements.
Year | Author | N | Reference | Comments |
---|---|---|---|---|
Accuracy of cardiac mass measurements | ||||
1986 | Keller | 10 | 219 | Animals; MRI LV mass in normal dogs; high accuracy versus autopsy |
1986 | Florentine | 11 | 220 | Animals; MRI LV mass in normal dogs and cats; high accuracy versus autopsy and reproducible |
1987 | Caputo | 13 | 221 | Animals; MRI LV mass in normal (7) and hypertrophied LV (6) dogs; high accuracy versus autopsy and reproducible |
1987 | Maddahi | 9 | 222 | Animals; MRI LV mass in normal dogs, in-vivo and ex-vivo; high accuracy versus autopsy |
1988 | Katz | 10/40 | 223 | Cadaver hearts/Pts; high accuracy of ex-vivo MRI measurements/high in-vivo reproducibility |
1989 | Shapiro | 15 | 224 | Animals; MRI LV mass before and after MI in dogs; MRI mass accurate pre and post MI versus autopsy |
1992 | McDonald | 10 | 225 | Animals; MRI LV and RV mass in dogs; high accuracy versus autopsy, high reproducibility for RV and LV mass |
1993 | McDonald | 27 | 226 | Animals; MRI LV mass in dogs post MI model; postinfarct remodeling attenuation by nitrates detected by MRI |
1995 | Bottini | 6/34 | 227 | Cadaver hearts/HTN, HCM Pts; high accuracy of ex-vivo MRI/higher in-vivo reproducibility than echo |
1999 | Lorenz | 10/75 | 228 | Animals/Pts; high accuracy of MRI LV mass in dogs; Pts scanned to obtain mean and variation in normals |
Accuracy of cardiac volume measurements | ||||
1985 | Longmore | 20/20 | 229 | Normals/angina Pts; good correlation of MRI LV, RV stroke volumes, moderate correlation of MRI with x-ray VG |
1985 | Rehr | 15 | 230 | Cadaver hearts; good correlation of MRI LV volumes with cadaver heart casts volumes |
1987 | Sechtem | 10/5 | 231 | Normals; good correlation of MRI LV and RV stroke volumes, and with LV stroke volume by echo (n = 5) |
1995 | Helbing | 22/20 | 232 | Healthy and CHD children; good correlation of MRI LV and RV stroke volumes, and RV stroke volume with PA and tricuspid flow by phase contrast MRI |
1987 | Firmin | 10 | 233 | Normals; good correlation of MRI LV stroke volume with aortic flow by phase contrast MRI |
2000 | Bellenger | 52 | 234 | HF Pts (CHRISTMAS substudy); modest agreement of MRI LV volumes and function with Echo, radionuclide VG |
Reproducibility of cardiac mass and volume measurements | ||||
1990 | Semelka | 19 | 235 | DCM (11) and LVH (8) Pts; high reproducibility of MRI for LV mass, volumes, function |
1990 | Semelka | 11 | 236 | Normals; high reproducibility of MRI for LV mass, volumes, function |
1992 | Germain | 20 | 237 | Angina (6), HTN (6), CMP (3), valvular lesion (3), SVT (2) Pts; higher reproducibility of MRI versus M-mode Echo |
1993 | Yamaoka | 20 | 238 | Pts with various CV disorders; good correlation between MRI and CT for LV mass, high reproducibility of MRI |
1993 | Mogelvang | 30 | 239 | HTN (10), IHD (17), HCM (2), DCM (1) Pts; high reproducibility of MRI volumes and LV mass (11), moderate correlation between MRI LV mass and 2D Echo (13) |
1995 | Bogaert | 12 | 240 | Normals; higher reproducibility of MRI for LV volumes, mass, EF versus Echo |
1996 | Matheijssen | 7 | 241 | Pts with anterior MI; high reproducibility of MRI LV volumes, mass, EF |
1998 | Osterziel | 50 | 18 | DCM pts; MRI used as end point for randomized trial of growth-hormone treatment |
2000 | Bellenger | 20/20 | 17 | Normals/HF Pts; higher reproducibility of MRI for LV volumes, mass, EF versus Echo |
2002 | Grothues | 20/40 | 242 | Normals/LVH, HF Pts; higher reproducibility of MRI for LV volumes, mass, EF versus Echo |
2004 | Bellenger | 34 | 19 | HF Pts (CHRISTMAS substudy); MRI used as end point for randomized trial of carvedilol |
The goal of cine imaging is to capture a movie of the beating heart in order to visualize its contractile function. Typically, between 20 and 25 cine frames are acquired per cardiac cycle, with each frame comprising 35 to 45 ms. CineMRI may be acquired in either a real-time single-shot mode or via a segmented k-space data acquisition approach (see Fig. 23–7). Real-time cineMRI can be performed during free breathing and with minimal patient cooperation, making it ideal for children or patients who have difficulty following breathing instructions. Segmented cineMRI is performed during a breath-hold and offers substantial improvement in image quality, with superior spatial and temporal resolution compared with that of real-time imaging. Thus, in clinical practice, segmented imaging is usually preferred.22,23 In segmented acquisition, data are collected over multiple, consecutive heartbeats (typically 5-10). During each heartbeat, blocks of data (segments) are acquired with reference to ECG timing, which represent the separate phases or frames of the cardiac cycle. After the full acquisition, data from a given phase, collected from the multiple heartbeats, are combined to form the complete image of the particular cine frame.
Currently, the most common imaging engine for cineMRI is SSFP. The advantages over other sequences such as GRE include intrinsically high signal-to-noise ratio (SNR) and excellent blood-myocardium contrast that facilitates the identification of the endocardial border.24 For the core examination, a short-axis stack from the mitral valve plane through the apex and two-, three-, and four-chamber long-axis views are obtained. In general, the slice thickness is 5 to 6 mm.
With recent technical and protocol advances, adenosine stress perfusion MRI has changed status from a promising research tool to an everyday clinical test.25 In part because of this transition, CMR itself has changed from a modality that was used nearly exclusively for boutique indications, such as cardiac neoplasms and arrhythmogenic right ventricular cardiomyopathy, to one that is now considered a competitive first-line test for the most common indications, including the assessment of ischemic heart disease.26 Currently, in dedicated CMR clinical centers, perfusion stress-testing is often the fastest growing component of the clinical volume and can comprise nearly half of all referrals.27 For these reasons, we have incorporated perfusion stress testing into the core examination.
The goal of perfusion imaging is to create a movie of the transit of contrast media (typically gadolinium-based) with the blood during its initial pass through the left ventricular (LV) myocardium (first-pass contrast enhancement). Usually 4 to 5 short-axis views are obtained every heartbeat, with a total of 40 to 60 heartbeats consisting of the entire first-pass (Fig. 23–10). Although more views could be obtained every two heartbeats, this is not recommended unless tachycardia is present, because clinically, the benefit of improving LV coverage does not outweigh the detriment of halving the sampling frequency of the dynamic first-pass process.
Figure 23–10.
First-pass perfusion MRI image acquisition. Images are acquired serially at multiple slice locations (usually 4-5 short-axis views for left ventricular coverage) every heartbeat to depict the passage of a compact contrast bolus as it transits the heart. Example images of 1 slice location are shown at several representative time points: before arrival of contrast (frame 1); contrast in RV cavity (frame 12); contrast in LV cavity (frame 22); peak contrast in LV myocardium (frame 30), showing normal perfusion in the septum (open arrowhead) and abnormal perfusion in the inferolateral wall (solid arrowhead); and the contrast wash-out phase (frame 50).
A variety of pulse sequences are in use today for perfusion MRI, and the pace of development is rapid. Common imaging engines are SSFP, GRE, and GRE–echo planar imaging hybrid sequences (see Fig. 23–7). Virtually all sequences include a saturation prepulse modifier to provide T1 weighting and to accentuate regional differences in myocardial gadolinium concentration (see Fig. 23–5A). Because images are acquired in single-shot mode, a parallel imaging28,29 modifier is essential to speed imaging and allow adequate LV coverage as well as reduced motion artifacts.30 In general, image readout times more than ∼120 ms can lead to substantial motion artifacts in images acquired during periods of the cardiac cycle in which there is rapid LV motion.
The timeline for a comprehensive CMR stress test is displayed in Fig. 23–8. After scout and cine imaging, the patient table is pulled out partially to allow direct observation of the patient and full access; adenosine (140 μg • kg−1 • min−1) is then infused under continuous electrocardiography and blood pressure monitoring for at least 2 minutes before the initiation of perfusion imaging. The perfusion sequence is then applied by the scanner operator, resulting in automatic recentering of the patient back into the scanner bore and commencement of imaging. Gadolinium contrast (0.075-0.10 mmol/kg body weight) is then administered, followed by a saline flush (≈50 mL) at a rate of at least 4 mL/s via an antecubital vein. On the console, the perfusion images are observed as they are acquired, with breath-holding starting from the appearance of contrast in the right ventricular cavity. If the scanner software does not provide real-time image display, breath-holding should be started no more than 5 to 6 seconds after beginning gadolinium injection. Breath-holding is performed to ensure the best possible image quality (ie, no artifacts due to respiratory motion) during the initial wash-in of contrast into the LV myocardium. Once the contrast bolus has transited the LV myocardium, adenosine is stopped and imaging is completed 5 to 10 seconds later. Typically, the total imaging time is 40 to 50 seconds, and the total time of adenosine infusion is 3 to 3.5 minutes.
Before the rest perfusion scan, a waiting period of approximately 15 minutes is required for gadolinium to sufficiently clear from the blood pool. During this time, additional cine scans and/or velocity/flow imaging for valvular or hemodynamic evaluation can be performed. For the rest perfusion scan, an additional dose of 0.075 to 0.10 mmol/kg of gadolinium is given, and the imaging parameters are identical to the stress scan. Approximately 5 minutes after rest perfusion, delayed enhancement imaging (see next section) can be performed. The total scan time for a comprehensive CMR stress test, including cine imaging, stress and rest perfusion, and delayed enhancement, is usually well under 45 minutes.
Unlike vasodilator radionuclide imaging, in which adenosine is typically infused for 6 minutes (tracer injection∼at 3 minutes), stress perfusion MRI is performed using an abbreviated adenosine protocol (∼3 minutes) because the requirements for imaging are different.25 With radionuclide imaging, maintaining a vasodilated state for 2 to 3 minutes after tracer injection is necessary to allow time for tracer uptake into myocytes. In contradistinction, with MRI, currently available gadolinium media are inert, extracellular agents that do not cross sarcolemmal membranes,31 and vasodilation needs to be maintained only for the initial first-pass through the myocardium. Although severe reactions to adenosine are rare, a shortened protocol is relevant because moderate reactions that affect patient tolerability are relatively commonplace.32 A minimum 2-minute infusion duration was chosen on the basis of physiological studies in humans demonstrating that maximum coronary blood flow is reached, on average, 1 minute after the start of intravenous adenosine infusion (140 μg · kg−1 · min−1) and in nearly all patients by 2 minutes.33
Myocardial viability and infarction are simultaneously examined using the technique known as delayed enhancement MRI (DE-MRI).34-38 In the literature, DE-MRI is used interchangeably with late gadolinium enhancement CMR or delayed hyperenhancement imaging. Although at first glance, the use of DE-MRI appears to be primarily in those with coronary artery disease, new applications are steadily arising over a wide range of cardiovascular disorders. Thus DE-MRI is an essential component of the core examination.
The goal of DE-MRI is to create images with high contrast between abnormal myocardial tissue, which generally accumulates excess gadolinium (after intravenous administration), and normal tissue, in which gadolinium concentration is low. This is currently best achieved using a segmented, GRE imaging engine with inversion recovery prepulse modifier to provide very strong T1 weighting.34-38 A parallel imaging28,29 modifier can be used to shorten acquisition time. Imaging is performed approximately 5 minutes after rest perfusion imaging or 10 to 15 minutes after a one-time intravenous gadolinium dose of approximately 0.15 mmol/kg if stress-rest perfusion imaging is not performed. Short- and long-axis views in the identical planes used for cine imaging are obtained during repeated 6- to 10-second breath-holds. Data acquisition (readout period) is timed with the ECG in middiastole to minimize cardiac motion. Only every other heartbeat is used for data collection to allow for adequate recovery of longitudinal relaxation between inversion pulses (if bradycardia is present, imaging can occur every heartbeat).39
After an intravenous bolus, gadolinium distributes throughout the intravascular and interstitial space while simultaneously being cleared by the kidneys. In normal myocardium, where the myocytes are densely packed, tissue volume is predominately intracellular (∼75%-80% of the water space).40 Because gadolinium is unable to penetrate intact sarcolemmal membranes,31 the volume of distribution is small, and one can consider viable myocytes as actively excluding gadolinium media. In acute MI, myocyte membranes are ruptured, allowing gadolinium to passively diffuse into the intracellular space. This results in an increased gadolinium volume of distribution and thus increased tissue concentration compared with normal myocardium.41-43 Similarly in chronic infarction, as necrotic tissue is replaced by collagenous scar, the interstitial space is expanded and gadolinium tissue concentration is increased.43
Higher tissue concentrations of gadolinium lead to shortened T1 relaxation. Thus, when the parameters are set properly, T1-weighted sequences such as used for DE-MRI can depict infarcted regions as bright or hyperenhanced, whereas viable regions appear black or nulled (see Figure 23–6). One of the most important parameters to set correctly is the time between the inversion prepulse and data readout, known as the inversion time. The acronym commonly used is TI, but to avoid confusion with T1 (longitudinal relaxation time), we prefer the use of IT. Figure 23–5B demonstrates that to maximize contrast between infarcted and viable myocardium, IT should be set to when the relaxation curve of viable myocardium crosses zero. In general, once the optimal IT has been determined, no adjustment is necessary if DE-MRI is completed in approximately 5 minutes. However, it is important to keep in mind that gadolinium gradually washes out of viable myocardium, and IT will need to be adjusted upwards if DE-MRI is performed at multiple time points after contrast administration.39
Compared with other imaging techniques that are currently used to assess myocardial viability, an important advantage of DE-MRI is the high spatial resolution. With a standard implementation, a group of 10 hyperenhanced pixels (voxel resolution ∼1.9 × 1.4 × 6 mm) in a DE-MRI image would represent an infarction of 0.16 g, or a region one-thousandth of the LV mass.38 This level of resolution, which is more than 40-fold higher than single-photon emission computed tomography (SPECT), allows visualization of even microinfarcts that cannot be detected by other imaging methods.37,44
Recently an ultrafast, real-time version of DE-MRI has been developed that can acquire snap-shot images during freebreathing.45,46 This technique uses an SSFP imaging engine in single-shot mode with parallel imaging acceleration and provides complete LV coverage in less than 30 seconds. This technique could be considered the preferred approach in patients more acutely ill, unable to breath-hold, or with irregular heart rhythm. However, compared with standard, segmented DE-MRI, sensitivity for detecting MI is mildly reduced, and the transmural extent of infarction may be underestimated.46
In the core examination, cardiac and proximal vascular structures are commonly assessed by SSFP cineMRI. Occasionally, additional structural/anatomical information is necessary to fully investigate the clinical question, such as in the setting of congenital heart disease, cardiac masses, or patients with aortic root dilation on initial three-chamber cineMRI. In general, a morphology scan consists of a series of parallel slices, which bread-loaf the anatomical region of interest (see Fig. 23–29A). Although any orientation may be imaged, usually axial, sagittal, or coronal planes (or all three) are chosen first.
In order to quickly provide substantial anatomical coverage, morphology imaging is primarily performed in single-shot mode using either an SSFP or turbo spin echo (TSE) imaging engine. The SSFP sequence is similar to that used for real-time cineMRI, but it has been altered to produce a stack of images that progresses through space rather than a cine movie loop at a single location. In its native form (without additional modifiers), SSFP produces images in which blood in the cardiac chambers and vasculature appear bright; thus it is known as a bright-blood technique. To first order, SSFP images are T2/T1 weighted.36 In contrast, spin-echo–based sequences such as TSE produce images in which flowing blood is dark; thus these are known as black-blood techniques. However, blood signal suppression may be incomplete, and a black-blood modifier, which consists of a double-inversion prepulse,47 is often added to improve blood nulling. A single-shot version of TSE that is commonly used in cardiovascular imaging is black-blood HASTE.
With SSFP or HASTE morphologic imaging, the entire thorax can be imaged in multiple orthogonal views in less than 2 minutes without breath-holding. The choice between SSFP and HASTE is made depending on the clinical question and whether bright- or black-blood contrast is desired. Occasionally, small structures may be obscured on SSFP imaging by bright signal from the blood. Conversely, stagnant blood flow by virtue of incomplete suppression may be mistaken as tissue on HASTE imaging. Accordingly, both techniques should be performed when images from one are inconclusive, because the time cost is minimal. If higher spatial resolution is desired for certain key views, segmented cineMRI or segmented TSE can be performed during a breath-hold. Black-blood sequences should be performed before gadolinium administration because shortening blood T1 will impair the suppression of blood signal. SSFP sequences can be performed either before or after gadolinium administration.
Depending on the clinical question, the core examination may include velocity-encoded cine imaging (VENC-MRI) to measure blood velocities and flows in arteries and veins and across valves and shunts. Also known as phase-contrast velocity mapping, the underlying principle is that signal from moving blood or tissue will undergo a phase shift relative to stationary tissue if a magnetic field gradient is applied in the direction of motion.
The goal of VENC-MRI is to produce a cine loop across the cardiac cycle, where on any given frame, pixel intensity is proportional to blood velocity. Generally displayed using a grayscale, white corresponds to maximum flow in one direction, black to maximum flow in the opposite direction, and mid-gray indicates that flow is absent (Fig. 23–11). Although blood velocity can be measured in any arbitrary direction, it is usually assessed in reference to the imaging plane. Encoding velocity in the slice gradient direction allows measurement of through-plane velocities, and encoding in either the frequency or phase-encode gradient directions allows in-plane measurement of velocity components directed either vertically or horizontally within the image plane.
Figure 23–11.
ECG gated velocity-encoding MRI. Analogous to cine imaging, each velocity-encoded image (top row of images) corresponds to a cardiac phase, and gating to the electrocardiogram is necessary. On the images, white represents maximal velocity (in this case across the aortic valve, red arrow). Black represents flow in the opposite direction (in the descending thoracic aorta, blue arrow). Grey represents no flow. The bottom row demonstrates the corresponding cineMRI images.
VENC-MRI is commonly performed using a segmented GRE imaging engine during a patient breath-hold. The sequence, however, is modified to measure the effects of a magnetic field gradient on the precessing protons within flowing blood. The precise details of VENC-MRI are complex and are considered more comprehensively elsewhere.48-50 However, optimizing the maximum velocity that can be measured—which is inversely related to gradient strength (for a constant application time)—is important. Setting the maximum velocity too low will lead to aliasing, whereas setting it too high will lead to more noise or inaccuracy in the velocity measurement. Retrospective rather than prospective ECG gating is preferred to allow data collection throughout the entire cardiac cycle, including end-diastole.
Although VENC-MRI appears analogous to Doppler echocardiography, there are important differences (Table 23–2). For instance, an advantage of VENC-MRI is that blood flow through an orifice is directly measured on an enface image of the orifice with through-plane velocity encoding. With echocardiography, there are two limitations. First, the blood flow profile is not directly measured but assumed to be flat (ie, velocity in the center of the orifice is the same as near the edges) so that, hopefully, one sampling velocity would indicate average velocity. Second, the cross-sectional area of the orifice is estimated from a diameter measurement of the orifice at a different time from when Doppler velocity was recorded using a different examination (M-mode or two-dimensional imaging). On the other hand, VENC-MRI has some disadvantages. Perhaps most importantly, VENC-MRI is not performed in real time and requires breath-holding to minimize artifacts from respiratory motion. One consequence is that it is difficult to measure changes in flow that occur with respiration.
Imaging Characteristic | Velocity-Encoding MRI | Doppler Echocardiography |
---|---|---|
Imaging during free breathing | Limited | Yes |
Imaging during arrhythmias | Limited | Yes |
Temporal resolution | ∼50 msa | <10 ms |
Peak velocity location | Yes | Location ambiguity (CW Doppler) |
Angle dependence | Yes, 20 degrees | Yes, 20 degrees |
Imaging planes | Any | Echocardiographic windows |
Blood flow profile | Directly measured | Flat profile assumed |
Flow quantification | En face | In-planeb |
T2-weighted imaging has shown promise in assessing acute, inflammatory processes.51 In the setting of myocardial necrosis, such as in acute MI or myocarditis, tissue myocardial water content increases substantially within the region of necrosis.52 The presence of tissue edema results in a longer intrinsic T2 for infarcted myocardium (60-65 ms) compared with that of normal (45-50 ms).53 Thus the goal of T2-weighted (T2W) imaging is to utilize the differences in T2 between normal and necrotic myocardium to create tissue contrast.
Currently, for edema imaging, many institutions use a TSE imaging engine with a black blood modifier (eg, a double inversion pulse). T2 weighting is achieved by using a long echo time (TE), typically approximately 50 to 80 ms. A variant, which adds T1 weighting via a third inversion pulse, is also commonly used and is known as short Tau inversion recovery (STIR). With STIR, there may be improved contrast between edematous and normal myocardium and more uniform fat saturation; however, SNR is worse and artifacts (see below) are more common. The choice of other parameters, including slice thickness, use of the body coil rather than the chest coil, analysis method, and so forth, are often institution-specific, and there is no consensus protocol. With appropriate settings, T2W-TSE or STIR can depict edematous regions as bright or hyperintense, whereas nonedematous regions appear grey.
One important role of T2W imaging may be in distinguishing chronic lesions from those of recent onset.51,54,55 For example, Abdel-Aty et al51 initially reported that STIR, when used in conjunction with DE-MRI, could accurately distinguish acute (<2 weeks) from chronic MI (>4 weeks) in >90% of cases. However, more recently, Kellman et al53 reported that interpretation of T2W-TSE is complicated by the frequent presence of artifacts that mimic edema and found its accuracy to be substantially lower due to a large number of false positives in patients with chronic MI. Typical artifacts include hyperintense slow flow artifacts and myocardial signal drop-out.53 The latter may be a result of a misalignment between the location of the slice selective reinversion (a component of the dark blood modifier) and the image readout.
Recent studies have suggested that another potential role of T2W-TSE/STIR is to define the entire region of hypoperfused myocardium associated with acute MI.56-58 These studies have suggested that, whereas DE-MRI identifies the area of irreversibly injured myocardium, the hyperintense regions on T2W imaging represent both irreversibly injured myocardium and myocardium that has been salvaged by reperfusion therapy—for example, the area at risk. The hypothesis that T2W imaging measures the area at risk is largely based on the finding that the extent of hyperintense regions on T2W-TSE/STIR are larger than the infarct size measured on delayed enhancement imaging.56,57 Unfortunately, the degree to which TSE/STIR imaging artifacts (as discussed above) confound the measurement of the hyperintense regions has not been systematically studied, and the use of T2W-TSE for identifying the risk region requires further validation.
Clinical Applications
The strength of CMR is its ability to evaluate pathologic processes using several pulse sequences—each tuned to different biological properties—during a single examination. However, the multitude of pulse sequences, in turn, makes available multiple approaches to assessing the clinical question. In this section, we overview common approaches discussed in the CMR literature. Technical feasibility is reviewed, but placed in context with the practical concerns of a busy clinical CMR service, and optimal strategies are highlighted. We focus on common indications that are encountered in a general CMR practice, except vascular disease, which is discussed elsewhere (see Chap. 24). The evaluation of patients with coronary heart disease, heart failure, and cardiomyopathies is emphasized.
A number of CMR approaches exist for the detection of coronary artery disease (CAD). Each of these techniques exploit specific anatomical or physiological properties that occur as a result of CAD (Table 23–3). However, clinically, the most widespread approach for evaluating CAD is stress testing with imaging of either myocardial contraction or perfusion.
Physiologic Substrate | CMR Technique | Stress Testa | Clinical Application |
---|---|---|---|
Coronary anatomy and morphology | Coronary MRA | None | +b |
Coronary artery blood flow/velocity | Velocity encoding MRI | Adenosine/dipyridamole | −c |
Deoxygenated hemo-globin content | BOLD MRI | Adenosine/dipyridamole | − |
Phosphocreatinine/ATP content | 31P-Spectroscopy | Handgrip exercise | − |
Ventricular function | Multiphase cineMRI | Dobutamine | + + |
Myocardial perfusion | Dynamic first-pass perfu-sion contrast-enhanced MRI | Adenosine/dipyridamole | + + |
Analogous to echocardiography, cineMRI during dobutamine stimulation can be used to detect ischemia-induced wall-motion abnormalities. Dobutamine cineMRI may yield higher diagnostic accuracy than dobutamine echocardiography59 and can be effective in patients not suited for echocardiography as a result of poor acoustic windows.60 Recent data suggest that the presence of inducible ischemia by this technique predicts subsequent cardiac mortality.61-65 As a result, the American College of Cardiology has specified that the use of dobutamine cineMRI is appropriate for the detection of CAD in symptomatic patients with intermediate pretest probability, who have uninterpretable ECGs or are unable to exercise.26 Nonetheless, a major practical issue is the need to administer dobutamine while the patient is inside the magnet. Inotropic stimulation in patients with ischemic heart disease is intrinsically associated with the risk of eliciting an ischemic event, and the position of the patient within the magnet impairs physician–patient interaction. In addition, the diagnostic utility of the ECG is diminished during imaging because the shape of the ECG waveform is directly altered by the magnetic field. Thus, from a practical standpoint, there are many logistical issues regarding patient safety and adequate monitoring that require thorough planning and experienced personnel. Perhaps in part, because of these issues, only a few centers worldwide routinely perform this technique.
First-pass perfusion MRI during adenosine vasodilation is rapidly becoming the stress test of choice in clinical CMR centers. Perfusion MRI is promising for several reasons. Decreased perfusion is the first step in the ischemic cascade. Therefore, techniques that assess perfusion have the potential to be more sensitive than techniques that assess later steps.66-70 Logistically, as discussed earlier (see Fig. 23–8), stress perfusion imaging is quick and simple. The duration of adenosine infusion is short (∼3 minutes), and direct access to the patient is limited only during imaging of the first pass (∼45 seconds). Compared with competing technologies such as radionuclide imaging, perfusion MRI has many potential advantages: more than an order of magnitude improvement in spatial resolution (typical voxel dimensions, MRI 3.0 × 1.8 × 8 mm = 43 mm3 vs SPECT 10 × 10 × 10 mm =1000 mm3; Fig. 23–12); the ability to identify regional differences in flow over the full range of coronary vasodilation (ie, no plateau in signal at high flow rates, as seen with radionuclide tracers71,72; Fig. 23–13); the lack of ionizing radiation; and an examination time of 30 to −45 minutes versus 2 to 3 hours.
Figure 23–13.
Comparisons of perfusion MRI, radionuclide, and microsphere flows. MRI signal intensity-time curves were linearly related to reference microsphere flows over the full range of vasodilation. Relationships between technetium-99m (99mTc-sestamibi) and thallium-201 (201Tl) activity and microsphere flows were curvilinear, plateauing as flows increased. Data suggest that perfusion MRI, unlike radionuclide imaging, has the potential for detecting stenoses producing only moderate limitations in flow reserve. A. Normalized magnetic resonance first-pass perfusion (MRFP) imaging and full-thickness microsphere relative regional flows (RRF). B. Normalized 99mTc-sestamibi and full-thickness microsphere RRFs. C. Normalized 201Tl and full-thickness microsphere RRFs. D. In-vivo SPECT and ex-vivo well-counting values of 99mTc-sestamibi versus microsphere RRFs. Reproduced with permission from Lee et al.70
The diagnostic performance of stress perfusion MRI has been evaluated in a number of studies in humans.25,73-90 Overall, these studies have shown good correlations with radionuclide imaging and x-ray coronary angiography. Table 23–4 summarizes the published stress perfusion MRI studies in humans with coronary angiography comparison. A total of 34 studies have been completed, consisting of more than 2800 patients with known or suspected CAD. On average, the sensitivity and specificity of perfusion MRI for detecting obstructive CAD were 85% and 81%, respectively. The American College of Cardiology16 has indicated that the use of stress perfusion MRI is appropriate for detection of CAD in symptomatic patients with intermediate pretest probability and who have uninterpretable ECGs or are unable to exercise.26
Year | Author | Reference | No. of Patients | Patients With Known CAD Excluded | MRI Perfusion Protocola | Gadolinium Dose (mmol/kg) | Pulse-Sequence | X-Ray Angiography (CAD definition) | Analysis Methodb | Sensitivity | Specificity |
---|---|---|---|---|---|---|---|---|---|---|---|
1993 | Klein | 75 | 5 | No | Stress | 0.05 | IR-GRE | >50 | Prospective | 81c | 100c |
1994 | Hartnell | 76 | 18 | No | Rest/Stress | 0.04 | IR-GRE | ≥70 | Prospective | 83 | 100 |
1994 | Eichenberger | 78 | 10 | No | Rest/Stress | 0.05 | GRE | >75 | Retrospective | 44c | 80c |
2000 | Al-Saadi | 77 | 34 | Yes | Rest/Stress | 0.025 | IR-GRE | ≥75 | Prospectived | 90 | 83 |
2001 | Bertschinger | 79 | 14 | No | Stress | 0.1 | SR-EPI | ≥50 | Retrospective | 85 | 81 |
2001 | Schwitter | 80 | 48 | Yes | Stress | 0.1 | SR-GRE-EPI | ≥50 | Retrospective | 87 | 85 |
2001 | Panting | 81 | 22 | No | Rest/Stress | 0.05 | IR Spin Echo-EPI | >50 | Retrospective | 79 | 83 |
2002 | Sensky | 82 | 30 | No | Rest/Stress | 0.025 | IR-GRE | >50 | Prospective | 93c | 60c |
2002 | Ibrahim | 83 | 25 | No | Rest/Stress | 0.05 | SR-GRE-EPI | >75 | Retrospective | 69c | 89c |
2003 | Chiu | 84 | 13 | Noe | Rest/Stress | 0.05 | IR-SSFP | >50 | NS | 92c | 92c |
2003 | Ishida | 85 | 104 | No | Stress/Rest | 0.075 | SR-GRE-EPI | ≥70 | Prospective | 90 | 85 |
2003 | Nagel | 86 | 84 | No | Rest/Stress | 0.025 | SR-GRE-EPI | ≥75 | Retrospective | 88 | 90 |
2003 | Doyle | 87 | 138 | No | Rest/Stress | 0.04 | SR-GRE | ≥70 | Prospectived | 57 | 85 |
2004 | Wolff | 88 | 75 | No | Stress/Rest | 0.05-0.15 | SR-GRE-EPI | ≥70 | Prospectivef | 93 | 75 |
2004 | Giang | 89 | 80 | No | Stress | 0.05-0.15 | SR-GRE-EPI | ≥50 | Retrospectivef | 93 | 75 |
2004 | Paetsch | 90 | 79 | No | Stress/Rest | 0.05 | SR-GRE-EPI | >50 | Prospective | 91 | 62 |
2004 | Thiele | 243 | 20 | No | Rest/Stress | 0.05 | SR-GREg | ≥70 | Retrospective | 75 | 97 |
2004 | Plein | 73 | 68 | Noe | Rest/Stress | 0.05 | SR-GRE6 | ≥70 | Prospective | 88 | 83 |
2005 | Plein | 74 | 92 | No | Rest/Stress | 0.05 | SR-GRE6 | >70 | Retrospective | 88 | 82 |
2005 | Sakuma | 244 | 40 | Yes | Rest/Stress | 0.03 | SR-GRE | ≥70 | Prospective | 81 | 68 |
2006 | Klem | 25 | 92 | Yes | Stress/Rest | 0.065 | SR-GRE6 | ≥70 | Prospective | 84g | 58g |
2006 | Cury | 245 | 47 | No | Stress/Rest | 0.1 | SR-GRE-EPI | ≥70 | Prospective | 81ch | 87ch |
2006 | Rieber | 246 | 43 | No | Stress/Rest | 0.05 | SR-GRE | >50 | Retrospective | 88 | 90 |
2006 | Pilz | 247 | 171 | No | Stress/Rest | 0.1 | SR-GRE-EPI | >70 | Prospective | 96 | 83 |
2007 | Greenwood | 98 | 35 | Noe | Rest/Stress | 0.05 | SR-GREi | ≥70 | Prospective | 86j | 100 j |
2007 | Cheng | 248 | 61 | No | Stress/Rest | 0.04 | SR-GREi | ≥50 | Prospective | 90 | 67 |
2007 | Costa | 249 | 30 | No | Stress/Rest | 0.1 | SR-GRE | ≥50 | Retrospective | 85c | 39c |
2008 | Burgstahler | 250 | 20 | Yesk | Stress/Rest | 0.1 | SR-SSFPi | >70 | Prospective | 100 | 80 |
2008 | Klein | 251 | 54 | Yes | Stress/Rest | 0.05 | SR-SSFP | >50 | Prospective | 87 | 88 |
2008 | Doesch | 96 | 141 | No | Stress/Rest | 0.1 | SR-GREi | ≥75 | Prospective | 90j | 77j |
2008 | Schwitter | 252 | 234l | No | Stress/Rest | 0.1l | SR-GRE | ≥50 | Prospective | 85 | 67 |
2008 | Klem | 94 | 80 | Yes | Stress/Rest | 0.07 | SR-GRE | ≥70 | Prospective | 86j | 91j |
2008 | Kitagawa | 95 | 50 | No | Stress/Rest | 0.05 | SR-SSFP | ≥50 | Prospective | 86g,m | 75g,m |
2009 | Bernhardt | 97 | 823 | No | Stress | 0.1 | SR-GRE-EPI/-SSFP | ≥70 | Prospective | 89g,n | 81g,n |
Total | 34 | 2880 | |||||||||
Average | 85 | 81 |
Despite the mostly favorable results of these studies, a number of issues should be considered. Some studies are of limited clinical applicability because they required central venous catheters,77 imaged only one slice per heartbeat,77,81 or excluded patients with diabetes.86 Many studies had small sample sizes—11 studies had 30 or fewer patients. Most included patients already known to have CAD or known to have prior MI. In these studies, there is pretest referral or spectrum bias, which can artificially raise test sensitivity and/or specificity.91,92 Importantly, in many studies after the data were collected, several methods of analysis were tested, and different thresholds for test abnormality were appraised. For these studies, the reported sensitivity and specificity values are optimistic because the end points were chosen retrospectively and they represent optimized values.
Several practical issues should also be considered when evaluating these studies. The studies in Table 23–4 are fairly heterogeneous in terms of the protocols and methods used. In part, this is a reflection of the rapid pace of pulse sequence development over this time frame. Thus earlier studies may have used pulse sequences and/or imaging protocols that are no longer commonly used in a clinical setting, and some reports may not be directly comparable. For instance, the dose of gadolinium contrast administered has been variable (over a six-fold range—0.025-0.15 mmol/kg). Second, several studies used a quantitative approach (ie, regions-of-interest are drawn on the images and image intensities are measured) for diagnostic assessment. Although a quantitative approach has the potential advantage of allowing the measurement of absolute blood flow, the approach requires extensive interactive postprocessing and is time-consuming. As a result, a quantitative approach has not been feasible for general clinical use and is uncommonly used outside of the research setting.
In contrast, image interpretation by simple visual assessment would be a realistic approach for a clinical CMR practice. Unfortunately, the results in the literature regarding visual assessment of perfusion MRI are mixed, generally demonstrating adequate sensitivity but relatively poor specificity for the detection of CAD. In large part, image artifacts are responsible for reduced specificity. However, there is no reason to interpret the stress perfusion images in isolation. The core examination is a multicomponent protocol that includes cine and delayed-enhancement imaging in addition to stress and rest perfusion. In this context, it is noteworthy that an interpretation algorithm that combines data from perfusion MRI and DE-MRI has been introduced that substantially improves the specificity and accuracy of rapid visual assessment for the detection of CAD (Fig. 23–14).25
Figure 23–14.
Interpretation algorithm for incorporating delayed enhancement imaging (DE-MRI) with stress and rest perfusion MRI for the detection of coronary disease. A. Schema of the interpretation algorithm. (1) Positive DE-MRI study: Hyperenhanced myocardium consistent with a prior myocardial infarction (MI) is detected. Does not include isolated midwall or epicardial hyperenhancement, which can occur in nonischemic disorders. (2) Standard negative stress study: No evidence of prior MI or inducible perfusion defects. (3) Standard positive stress study: No evidence of prior MI, but perfusion defects are present with adenosine that are absent or reduced at rest. (4) Artifactual perfusion defect: Matched stress and rest perfusion defects without evidence of prior MI on DE-MRI. B. Patient examples. (top row) Patient with a positive DE-MRI study demonstrating an infarct in the inferolateral wall (red arrow) although perfusion-MRI is negative. The interpretation algorithm (step 1) classified this patient as positive for coronary artery disease (CAD). Coronary angiography verified disease in a left circumflex (LCX) marginal artery. CineMRI demonstrated normal contractility. (middle row) Patient with a negative DE-MRI study but with a prominent reversible defect in the anteroseptal wall on perfusion-MRI (red arrow). The interpretation algorithm (step 3) classified this patient as positive for CAD. Coronary angiography demonstrated a proximal 95% left anterior descending (LAD) stenosis. (bottom row) Patient with a matched stress-rest perfusion defect (blue arrows) but without evidence of prior myocardial infarction on DE-MRI. The interpretation algorithm (step 4) classified the perfusion defects as artifactual. Coronary angiography demonstrated normal coronary arteries. From Klem I, Heitner JF, Shah DJ, et al. Improved detection of coronary artery disease by stress perfusion cardiovascular magnetic resonance with the use of delayed enhancement infarction imaging. J Am Coll Cardiol. 2006 Apr 18; 47(8):1630-1638.
The algorithm is based on two simple principles. First, with perfusion MRI and DE-MRI, there are two independent methods to obtain information regarding the presence or absence of MI. Thus one method could be used to confirm the results of the other. Second, DE-MRI image quality (eg, signal-to-noise ratio) is far better than perfusion MRI because it is less demanding in terms of scanner hardware (DE-MRI images can be built up over several seconds rather than in 0.1 seconds as is required for first-pass perfusion).93 Thus DE-MRI should be more accurate for the diagnosis of MI.93 Conceptually, it then follows that perfusion defects that have similar intensity and extent during both stress and rest (matched defect) but do not have infarction on DE-MRI are artifactual and should not be considered positive for CAD. Conversely, the presence of infarction on DE-MRI favors the diagnosis of CAD even if the results of perfusion imaging are equivocal.
This multicomponent approach to image interpretation has been studied in a variety of patient cohorts, including those at intermediate risk for CAD,25,94 with known or suspected CAD,95,96 with history of revascularization,97 and undergoing risk stratification after successful thrombolytic therapy for ST-segment elevation MI (STEMI).98 Although direct head-to-head data are lacking, these studies suggest that rapid visual assessment using this combination protocol provides results that are comparable to, if not better than, competing technologies. As a result, many clinical laboratories have adopted this methodology.
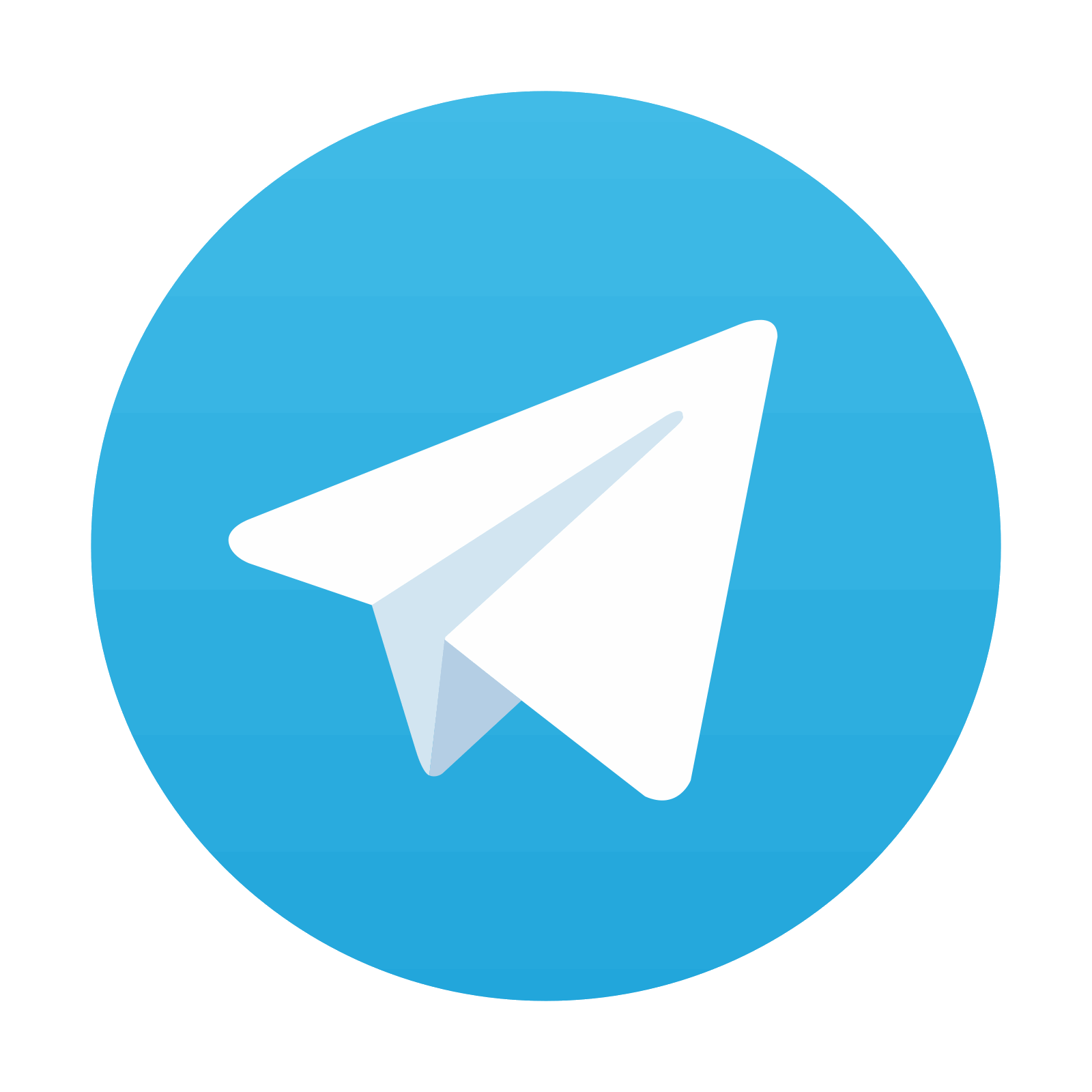
Stay updated, free articles. Join our Telegram channel
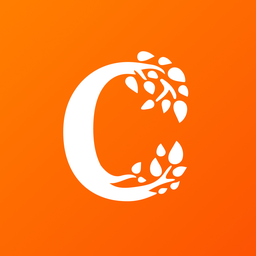
Full access? Get Clinical Tree
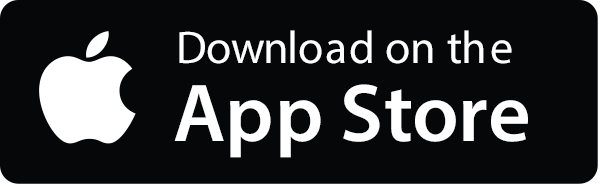
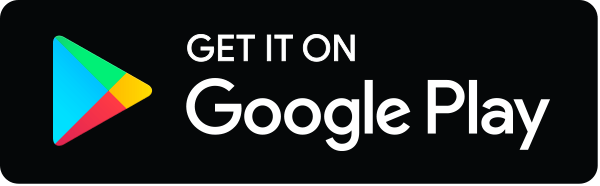