Introduction
The pathogenesis of many pulmonary diseases involves the processes of lung injury and repair, and indeed maintenance of normal lung homeostasis involves cycles of ongoing subclinical microinjury and repair. Injury can be viewed at different levels from the context of whole-organ function or at the level of individual cells or molecules. Similarly, repair can be considered at several levels. Repair can be considered as a sequence of pathologic processes as in repair of a skin wound, at the level of cell replacement, at the level of patching of holes in plasma membranes, or at the level of molecules broken down in the proteosome and replaced by de novo synthesis. Given the complexity of these processes, we provide our definition of the terms injury and repair with the aim of limiting what would otherwise be an exhaustive discussion. In this chapter we will focus on the cellular injury resulting in the dysfunction or death of cells that underlies the pathogenesis of many pulmonary diseases. Injury to any of the different cell types residing in the lung can ultimately lead to organ dysfunction (for a review of lung structure, see Chapter 1 ). A broad concept of lung repair includes processes by which the function of the injured lung is restored to normal. In regard to reparative processes, we will focus on the cellular aspects of repair, including replacement of destroyed cells and restoration of normal cellular function.
Lung Injury and Repair during Homeostasis
As a major portal to the environment, the lung is continuously exposed to a vast array of chemical and biologic agents that can cause cellular dysfunction or even death. Acute episodes of injury to the lung, as a result of viral infection or chemical exposures, result in transient alterations in lung function. Repair therefore can be defined as restitution of the cells, and thus of the lung, to the preinjury level of structure and function. Normal repair returns the lung to a healthy state that is capable of responding to subsequent injuries. The response of the lung to repeated injury and repair is shown in Figure 15-1 . Normal aging is manifest by steady and progressive declines in cell number and/or function and in overall lung function ( Fig. 15-1 , straight blue line), in part due to episodes of recurrent microinjury and decreased capacity of the epithelium or endothelium to heal itself. Normal repair can return the lung function to its prior state ( Fig. 15-1 , fluctuating blue line). Faulty repair leads to episodic loss of function that ultimately results in respiratory failure ( Fig. 15-1 , orange line).

Lung Injury and Repair during Disease
Many common pulmonary disorders, such as chronic obstructive pulmonary disease (COPD), interstitial lung disease, asthma, cystic fibrosis, and the acute respiratory distress syndrome (ARDS), are characterized by cellular injury and faulty repair resulting in clinically significant physiologic abnormalities. The intensity and duration of the insult contribute to the time of onset of symptoms and the chronicity of disease. In ARDS, a severe insult causes widespread lung injury and respiratory failure in hours to days. By contrast, in COPD, years of exposure to the offending agent (usually cigarette smoke in the developed world and biomass fuels in developing countries) results in slowly progressive loss of respiratory function. Patients with interstitial lung disease often have a stuttering course with periods of rapidly declining lung function interspersed with periods of relative quiescence. Importantly, in addition to injury, dysfunctional repair and “remodeling” contribute to the pathogenesis and clinical course of lung diseases, including COPD, asthma, interstitial lung disease, and fibroproliferative ARDS.
Lung Injury
The pathogenesis of many lung diseases involves an exogenous (e.g., inhaled toxin, allergen, infectious agent) or endogenous (inflammation or autoimmune) agent causing cellular dysfunction or death. In COPD, inhaled toxins such as those that make up cigarette smoke initiate processes that culminate in epithelial and endothelial cell death, as well as destruction of extracellular matrix, the scaffolding of the lung. In asthma, allergens, environmental pollutants, pathogens, and the inflammatory response to these agents induce injury to the bronchial epithelium. Pulmonary fibrosis is thought to reflect repetitive injury to the lung epithelium interspersed with periods of relative quiescence.
To illustrate the processes involved in lung injury, we will focus on inflammatory lung injury in ARDS. An extensive review of the causes, pathogenesis, clinical manifestations, and treatment of ARDS is included in Chapter 100 . Briefly, ARDS is defined as the acute onset (within 1 week of the inciting event) of hypoxemia and bilateral opacities on chest imaging attributable to noncardiogenic (increased permeability) pulmonary edema. The severity of ARDS is graded according to the ratio (“P/F ratio”) of the partial pressure of oxygen in the arterial blood to the fractional concentration of oxygen in the inspired air, with a P/F ratio of 200 to 300 defined as “mild,” 100 to 200 defined as “moderate,” and less than 100 defined as “severe.” ARDS is a heterogeneous syndrome rather than a single disease, and predisposing causes include pneumonia, extrapulmonary sepsis, inhalational injury, aspiration, injurious (high tidal volume) mechanical ventilation, pancreatitis, trauma, and blood transfusion. In addition to the acute medical condition, risk factors for the development of ARDS include acquired disorders such as alcohol abuse or cigarette smoking and genetic determinants such as certain genetic polymorphisms ( Fig. 15-2 ). Treatment of ARDS is mainly supportive and includes lung protective (low tidal volume) mechanical ventilation and a conservative fluid management strategy.

Epithelia and endothelia form selective barriers separating various body compartments of different composition from each other or the body from the environment. This barrier function depends on the presence of an intact layer of viable cells as well as the intercellular junctions that link adjacent cells, thus restricting the movement of fluid, ions, and macromolecules. In the healthy lung, the epithelium due to its tight intercellular junctions is the major barrier for passive fluid leak into the alveolar spaces, and, in addition, there is active fluid removal from the alveolar space via the action of specialized ion pumps and transporters in the epithelial cells. In ARDS, compromise of the selective barriers, epithelial as well as endothelial, either via disassembly of intercellular junctions or via death and sloughing of cells, results in an increase in lung permeability and the influx of protein-rich edema fluid, which leads to refractory hypoxemia and bilateral infiltrates on chest radiographs. Cellular dysfunction, such as impaired fluid transport and decreased surfactant production, further contributes to the impaired lung compliance and gas exchange abnormalities.
In ARDS, epithelial and endothelial injury is largely attributable to excessive and dysregulated inflammation ( Fig. 15-3 ) (see Chapter 100 ). In the case of pneumonia, the most common cause of ARDS, microbial products are recognized by resident lung cells, which in turn secrete chemoattractants that recruit inflammatory cells, initially neutrophils, into the lungs. Chemoattractants stimulate neutrophil actin assembly, resulting in stiffening and retention of neutrophils in the pulmonary capillaries. This is followed by intercellular adhesion molecule–mediated adhesion to the endothelium, a process that requires heparanase-mediated cleavage of the glycocalyx, egress from the bloodstream, and transmigration across the epithelium into the air spaces. Neutrophils contain potent antimicrobial compounds, including oxidants, proteinases, and cationic peptides. During immune surveillance or a normal immune response, neutrophils ingest (phagocytose) microorganisms and release these antimicrobial compounds directly into the phagosome. Neutrophil influx into the lungs under most circumstances does not result in tissue injury. However, during excessive and dysregulated inflammation in ARDS, large numbers of activated neutrophils release proteolytic enzymes and oxidants into the extracellular space, causing tissue injury. For example, neutrophil elastase, although inherently antimicrobial and critical for host defense, has been shown to cause injury not only to the extracellular matrix in COPD but also to the alveolar capillary membrane in ARDS. Importantly, neutrophil elastase can cause both a disruption of intercellular junctions and death of endothelial and epithelial cells. ARDS patients have elevated levels of elastase in their bronchoalveolar lavage fluid and plasma, and these levels correlate with the severity of lung injury. Unfortunately, pharmacologic agents that inhibit neutrophil elastase have not proven to be effective in the treatment of ARDS.

In addition to neutrophil elastase, other serine proteases, matrix metalloproteinases (MMPs) and cysteine proteinases are released by inflammatory cells and contribute to tissue destruction during lung injury. Increased levels of MMPs, derived from both neutrophils and macrophages, are present in patients with ARDS and contribute to lung injury. The mechanisms by which MMPs cause lung injury have not been fully elucidated. They are known to degrade junctional proteins in both epithelial and endothelial cells and induce cell death, although the latter is dependent on the cell type and specific MMP. Conversely, some MMPs promote survival of the lung epithelium, and others may attenuate injury or even promote repair.
Defensins are cationic peptides released from inflammatory cells that have potent antimicrobial properties. As with other inflammatory mediators, defensins are released into the extracellular space in ARDS and can cause lung injury, including endothelial and epithelial cell death and noncytotoxic injury. Similar to proteinases, in certain contexts, defensins may also promote lung repair.
In addition to proteinases and antimicrobial peptides, oxidants, including reactive oxygen and nitrogen species, are released by inflammatory cells during ARDS and contribute to tissue injury, including epithelial and endothelial cell death and disruption of tight junctions. Although the lung possesses potent endogenous antioxidant mechanisms, which serve to limit injury, in ARDS these mechanisms are overwhelmed by the large amount of reactive species generated. Additionally, oxidants can potentiate proteinase-induced lung injury, in part by inactivating antiproteinases. Finally, lipid mediators and another recently recognized antimicrobial weapon, neutrophil extracellular traps, can cause epithelial and endothelial injury.
Although much of the early injury to the alveolar capillary membrane is attributable to neutrophils (polymorphonuclear leukocytes) and their mediators, polymorphonuclear leukocytes cannot be the only perpetrators of lung injury because ARDS can develop in neutropenic patients. Recruitment of monocytes to the lungs follows the initial neutrophilic response in ARDS. In contrast to resident alveolar macrophages, which tend to have anti-inflammatory functions, recruited macrophages secrete proinflammatory mediators, including cytokines, chemokines, and lipids, that propagate the inflammatory response. Recruited macrophages also release toxic mediators, including reactive oxygen and nitrogen species, MMPs, tumor necrosis factor (TNF)-α, vascular endothelial growth factor, and interferon-β, which may enhance host defense but also induce tissue injury. In addition, recruited macrophages also play a critical role in both tissue repair and the resolution of inflammation (see later). The role of macrophages in lung inflammation, injury, and repair is further reviewed in Chapter 12 . Finally, in addition to neutrophils and macrophages, platelets, coagulation factors, products of infectious agents, inhaled toxins, oxygen, and mechanical forces all can contribute to injury to the alveolar capillary membrane. Indeed, a diverse array of mechanisms, mediators, and signaling pathways has been implicated in lung injury. Because the spectrum of injurious agents in ARDS is so broad, it is not surprising that pharmacologic strategies to block a single pathway or class of mediators have been ineffective.
Having reviewed the pathogenic agents that induce lung injury, we will now focus on exploring what constitutes lung injury. As mentioned earlier, we define injury as cellular dysfunction or death. Although cellular dysfunction in ARDS includes impaired production of surfactant, which is critical for lung compliance and important in host defense, we will focus here on the cellular dysfunction and death that contribute to increased lung permeability. In inflammatory lung injury the cellular dysfunction that contributes to edema formation includes (1) disruption of intercellular junctions that are responsible for maintaining the barrier function of the endothelium and epithelium and (2) impaired active fluid transport that is responsible for fluid reabsorption and maintenance of dry air spaces. Transient opening and closing of intercellular junctions is necessary for the transmigration of immune cells during immune surveillance or during a normal immune response and can take place without compromising barrier function. However, in ARDS, intercellular junctions of the endothelium and epithelium are disrupted, resulting in enhanced paracellular permeability, which contributes to the flooding of the alveolar spaces with edema fluid. This alveolar flooding is exacerbated by impaired function of the epithelial Na + /K + pumps and epithelial Na + channels that are responsible for fluid reabsorption. Attempts at stimulating fluid clearance with β-agonists have not improved outcomes in ARDS, perhaps because the barrier must be restored before the pumps can be effective (see Chapter 9 ).
In addition to the disruption of intercellular junctions, severe lung injury in ARDS and other lung diseases is characterized by cell death. In ARDS, inflammatory mediators induce death of endothelial cells as well as alveolar epithelial type I (ATI) cells, leaving surviving alveolar epithelial type II (ATII) cells, which are more resistant to injury, populating a largely denuded basement membrane. This dramatic injury to the alveolar epithelium engendered the term diffuse alveolar damage , which describes the histologic appearance of lungs taken from patients who died of ARDS.
Endothelial and epithelial cells can be lost via necrotic cell death or via programmed cell death, the latter termed “apoptosis.” Originally a morphologic definition, apoptosis might best be defined not only on the basis of nuclear condensation and characteristic DNA fragmentation but also based biochemically on activation of intracellular proteases termed “caspases.” Many recent reviews address the various mechanisms underlying apoptosis. However, it is important to note that there are two major pathways leading to apoptosis: the intrinsic pathway, involving signaling from the mitochondria; and the extrinsic pathway, deriving from signals generated from external stimulation of “death” receptors. In “normal” adult humans or experimental animals, snapshot analyses of lung tissue indicate only small scattered examples of replicating cells or of cells undergoing apoptosis. Because apoptotic cells are actively extruded from epithelial surfaces and cleared rapidly, the fact that evidence of apoptosis is detectable at all may indicate a higher degree of cellular turnover than previously thought. Early reports suggested that mitochondrial regulation of alveolar epithelial apoptosis may be induced by the proapoptotic BCL2 family member BAX and p53. Additional literature suggests that death receptors of the TNF receptor family such as FAS mediate apoptosis via the extrinsic pathway. Both FAS and FAS ligand levels are elevated in the edema fluid of ARDS patients, they induce epithelial cell apoptosis in a manner dependent on their modulation by oxidants and proteases, and elevated levels are predictive of worse clinical outcomes. In animal models of lung injury, FAS and TNF-related apoptosis-inducing ligand induce epithelial cell apoptosis, and inhibition of apoptosis improves survival. Conversely, hyaluronan, while promoting inflammation, attenuates epithelial cell apoptosis. Apoptotic epithelial cells are engulfed by both professional phagocytes (macrophages) and other epithelial cells.
In ARDS, lung cells can also die by necrosis, which leads to disruption of cell membranes with subsequent release of cellular contents that may be injurious. Causes of epithelial and endothelial necrosis in ARDS include physical effects of acid from inspiration of stomach contents, inhalation of toxic materials or fumes, infection with lytic viruses or bacterial products, hyperoxia, and mechanical disruption of cell membranes during mechanical ventilation. In sum, cell death is a major mechanism of lung injury, resulting in denudation and contributing to lung permeability and the massive influx of edema fluid, despite the existence of endogenous protective mechanisms.
Lung Repair
The ability of the lung to withstand considerable damage and repair itself enables recovery and survival from a variety of noxious stimuli. For the purpose of this chapter, we define repair broadly as processes by which the function of the injured lung is restored to normal . Repair of the lung after acute injury requires restitution of the endothelial and epithelial barriers, clearance of edema fluid, and the resolution of inflammation. In the case of ARDS, survival depends on repair of the injured lung, although some survivors do not regain normal lung function.
Endothelial Repair
Given that lung injury involves disruption of intercellular junctions and cell death, repair requires repopulation of the denuded basement membrane and reassembly of these junctions. Elegant work has demonstrated the importance of sphingosine-1-phosphate, released from activated platelets, in stimulating reassembly of endothelial intercellular junctions via Rho- and Rac-dependent cytoskeletal rearrangement. This process is dependent on αvβ3 integrin. In addition, the SLIT/ROBO mechanism was recently identified to stabilize the endothelial adherens junctions by promoting p120-catenin/E-cadherin association. Stabilization of actin cytoskeleton by the Rho GTPases enhances endothelial barrier integrity. Additional information on the regulation of endothelial function is found in Chapter 6 .
Epithelial Repair
Although the alveolar epithelium is more resistant to injury than is the endothelium, increased epithelial permeability is required for the development of airspace edema. Conversely, repair of the alveolar epithelium is critical for the resolution of edema, restoration of functions such as surfactant production and ion and fluid transport, and clinical outcome. In the epithelium, keratinocyte growth factor (KGF), interferon-γ, phosphatase and tensin homologue, epidermal growth factor, and c-Met are protective of tight junctional integrity during lung injury by mechanisms that involve cytoskeletal reorganization rather than enhanced expression of junctional proteins. Regulation of tight junctions in the alveolar epithelium after injury is an important and active area of investigation.
If the severity of the injury is such that there has been extensive cell death, the endothelium and epithelium must be repopulated. As an illustration of the mechanisms involved, we will focus on re-epithelialization of the denuded alveolar epithelium after injury. The injured alveolar epithelium re-epithelializes in stages, many of which are orchestrated by the ATII cell, the “defender of the alveolus.” These stages include (1) ATII cell spreading and migration, (2) ATII cell proliferation, (3) differentiation of ATII cells into ATI cells to restore a normal constitution of the alveolar epithelium, and finally (4) if the injury is extensive, migration and proliferation of bronchiolar stem cells to repopulate the injured alveolus ( Fig. 15-4 ; ). Recent work has identified various stem cell populations that likely contribute to this repair. Epithelial injury and repair promotes the activation of fibroblasts, which although important for physiologic wound repair, under certain circumstances, when dysregulated, can result in fibrotic lung disease. Each of these phenomena will be discussed in more detail in the following sections.

Cell Spreading and Migration
After death of alveolar epithelial cells, surviving ATII cells likely spread and migrate onto the denuded basement membrane. Although this has not been directly observed in the alveolar epithelium in vivo, cell spreading and migration is certainly the fastest mechanism for resealing the leaky epithelium based on in vitro observations and on observations of the importance of these phenomena in wound repair of other organs. Cell migration depends on tightly regulated assembly of the cytoskeleton leading to protrusion of “lamellipodia” and “filopodia” from the leading edge followed by contractile forces that release the rear edge from the extracellular matrix, processes that depend on the Rho GTPases. In the lung epithelium, cell spreading and migration after injury are triggered by soluble factors such as KGF, transforming growth factor (TGF)-α, TGF-β, interleukin-1β, and cytokine-induced neutrophil chemoattractant 2. Cell migration is further mediated by signaling pathways, including Rac1/TIAM1, phosphatase and tensin homologue, β-catenin, syndecan-1, adenosine triphosphate and dual oxidase 1, and vimentin, as reviewed elsewhere. Integrins, which are up-regulated in the alveolar epithelium after injury, contribute to cell migration via interactions with both the actin cytoskeleton and the extracellular matrix. The production of extracellular matrix as well as MMPs are critical to cell migration. MMPs enhance wound healing by cleaving cell-cell and cell–extracellular matrix adhesion molecules, activating chemokines and growth factors by proteolysis, and degrading the provisional matrix. Notably, cyclic stretch, imposed by mechanical ventilation during repair after ARDS, impedes cytoskeletal reorganization during cell spreading.
Cell Proliferation
In addition to cell spreading and migration, the denuded epithelial basement membrane is repopulated through the proliferation of surviving ATII cells, which accounts for the epithelial cell hyperplasia observed on histologic samples. In animal models, significant acute lung injury resulting in cell death triggers alveolar epithelial cell proliferation. Factors that promote ATII cell proliferation after injury include the heparin-binding growth factors KGF, hepatocyte growth factor, and heparin-binding epidermal growth factor, an effect that is significant enough to decrease mortality in animal models of lung injury, although the protective role of KGF may in part be attributable to enhanced epithelial cell survival. Other factors and pathways implicated in ATII cell proliferation after injury include granulocyte-macrophage colony-stimulating factor, which is also protective against injury, β-catenin signaling, macrophage migration inhibitory factor, and FOXM1. Proliferating ATII cells ultimately differentiate into ATI cells or die by apoptosis and are subsequently removed by macrophages or neighboring ATII cells. Mechanisms regulating differentiation of ATII cells into ATI cells are not well understood, but this process is promoted by TGF-β and insulin-like growth factor-I. The classic concept has been that ATII cells are responsible for alveolar repair by spreading into the denuded area, proliferating, and ultimately differentiating into ATI cells. However, at least in vitro, transdifferentiation is reversible. In this regard, a recent in vitro study reveals that ATI cells are also capable of cell spreading, migration, proliferation, and expression of surfactant protein-C, suggesting that these cells may also play a role in repair.
Role of Fibroblasts in Repair
Normal repair depends on epithelial-mesenchymal interactions, including the proliferation of subepithelial fibroblasts with deposition of granulation tissue. Mesenchymal cells provide signals and specific growth factors such as hepatocyte growth factor and KGF to epithelial cells to facilitate repair. By contrast, after repetitive and/or nonresolving injury, dysfunctional epithelial repair results in fibroblast recruitment, proliferation, and differentiation into myofibroblasts with deposition of excessive extracellular matrix composed of collagen and fibronectin. Severe or repetitive epithelial injury with dysfunctional repair can promote fibroproliferative responses in ARDS and other lung diseases, including idiopathic pulmonary fibrosis, asthma, and COPD, a topic which is reviewed in detail elsewhere. Although cytokines and growth factors that induce physiologic repair can prevent fibrosis under certain circumstances, under other circumstances, these same factors may actually promote fibrosis, underscoring the importance of context in lung repair. Therefore caution is indicated when considering therapeutic intervention aimed at accelerating repair of the injured lung, so as not to induce fibrosis. Epithelial “regeneration,” the restoration of normal lung architecture, must be distinguished from “simple repair,” which can include repopulation by abnormal cell types and scar formation.
Resolution of Inflammation
Ultimately, the inflammatory response must resolve in order to halt ongoing injury to the lung and allow reparative processes to proceed. An important step for resolution of inflammation is the apoptotic clearance of neutrophils. Neutrophils undergo apoptosis, a noninflammatory, nonimmunogenic form of cell death during which they retain their toxic mediators within their plasma membrane. This is in contrast to a necrotic cell death, in which neutrophils disintegrate with release of their intracellular constituents, including toxic and proinflammatory mediators, resulting in prolongation of the inflammatory response. Neutrophil apoptosis can be delayed by proinflammatory mediators such as granulocyte-macrophage colony-stimulating factor and endotoxin and by hypoxia, but it is enhanced by T regulatory lymphocytes in a TGF-β–dependent manner. Delayed neutrophil apoptosis is mediated by complex intracellular signaling pathways that involve hypoxia inducible factor-1 and cyclin-dependent kinases signaling through the antiapoptotic BCL2 family member MCL1. Apoptotic neutrophils are cleared by macrophages in a phagocytic process termed “efferocytosis” without release of toxic intracellular contents. Efferocytosis depends on the recognition of “eat me” signals, including phosphatidylserine and calreticulin, that are displayed on the apoptotic cell surface by a variety of receptors. This is followed by activation of Rho GTPases, leading to engulfment, which is regulated by the mitochondrial membrane protein UCP2, as well as HMGB1 and urokinase-type plasminogen activator. Efferocytosis results in the release of anti-inflammatory mediators that further promote the resolution of inflammation rather than inducing a proinflammatory macrophage response. Apoptotic neutrophils can also be efferocytosed by myeloid-derived suppressor cells in an interleukin-10–dependent manner. Whereas clearance of apoptotic neutrophils is usually highly efficient, yielding a low number of observable apoptotic cells at any given time, if apoptotic clearance is defective or overloaded, apoptotic neutrophils can undergo secondary necrosis or postapoptotic cytolysis. Thus, impaired neutrophil apoptosis and efferocytosis would prolong the duration of the inflammatory response, likely resulting in chronic inflammatory lung disease. Lipid mediators promote the resolution of inflammation by enhancing neutrophil apoptosis and efferocytosis, as well as through other mechanisms. In addition to neutrophils, inflammatory macrophages must also be cleared during the resolution of lung injury.
Stem Cells, Constitutive Cell Turnover, and Reparative Cell Types
Hierarchies of Reparative Cells
The concept of injury and repair is fundamentally linked to the stem cell attributes of mitotic potential and differentiation capacity. Mitotic potential has two components, the number of times a cell can undergo division and the time at which these cell divisions take place. Differentiation potential is defined in terms of the variety of differentiated cell types that can be generated by an individual mitotic cell. Many of the current concepts about stem and progenitor cell function in the lung derive from studies of airway epithelial cells. Comparatively less in known regarding the identity of long-term stem cells in the alveolar region and even less is known about resident stem and progenitor cells for the diverse lung mesenchymal cell populations. The knowledge of the stem and progenitor cells’ functions in the airway will be used as a foundation to illustrate the basic principles of how stem cells are involved in repair of the injured lung.
Classical Stem Cell Hierarchy
Within a classical stem cell hierarchy ( Fig. 15-5 ), the stem cell is defined as the reparative cell with the greatest mitotic and differentiation potential. A stem cell proliferates indefinitely and thus distributes its mitotic potential over the life span of the organism. These attributes of “stemness” are controlled by interaction of the stem cell with the stem cell microenvironment or niche. The niche serves to protect or sequester stem cells from factors that promote their differentiation. Stem cell division produces two daughter cells whose fate is determined by interactions with the stem cell niche. Symmetrical cell division results in both daughter cells retaining contact with the niche and maintenance of these nascent cells as stem cells. This mechanism, which results in amplification of stem cell number, has been demonstrated in tissues with rapid cell turnover, such as the bone marrow and gut. In contrast, asymmetric cell division results in generation of one daughter cell that maintains contact with the niche and is retained as a stem cell. The second daughter cell then loses contact with the niche and undergoes repeated cell division over a short period of time. This second daughter cell is the founding cell for the transit-amplifying cell population. Transit-amplifying cells are temporary constituents of the niche. They proliferate repeatedly over a short time period and then withdraw from the cell cycle as they commit to a differentiation pathway. Thus transit-amplifying cells serve to increase cell number and are destined to produce terminally differentiated cells. Terminally differentiated cells can have one or more phenotypes and constitute the majority of cells within a given tissue. These cells are largely responsible for conferring the phenotypic characteristics of a specific tissue or organ. Terminally differentiated cells are postmitotic. Thus loss of the terminally differentiated cell population acts as a stimulus for the differentiation of transit-amplifying cells that resupply this population.

Cells Involved in Lung Repair
Progenitor Cell
The term progenitor cell is a collective term used to describe any cell that has the capacity to proliferate. It is commonly used to indicate a cell that is in the process of cell division or has the potential to enter the cell cycle. The functional distinctions among lung progenitor cells are defined in the sections that follow.
Tissue-Specific Stem Cells
A tissue-specific stem cell is a rare cell type that is undifferentiated relative to its progeny. This cell has the capacity for unlimited self-renewal as a consequence of stable interactions with a stem cell niche. In the context of a hierarchy, the stem cell is defined as the reparative cell with the greatest mitotic and differentiation potential. Adult tissue stem cells have a differentiation potential equivalent to the cellular diversity of the tissue in which they reside. Because of the relatively quiescent state of the pulmonary epithelium, lung stem cells are identified as cells that are activated in response to severe cell depletion. As a consequence of this experimental approach, we will review the properties of lung stem cells in the context of severe injury.
Tracheobronchial tissue-specific stem cells have been evaluated in detail in mice. These cells exhibit a low cuboidal to pyramidal morphology and are termed basal cells. Additional studies have suggested that the tracheobronchial tissue-specific stem cell resides within specialized microenvironments located at the submucosal gland duct junction and in intercartilaginous regions of the trachea and bronchial epithelium. In murine bronchioles, tissue-specific stem cells have been identified within neuroepithelial bodies and bronchiolar duct junction microenvironments. These cells are resistant to club cell (Clara)–specific toxicants such as naphthalene and express the molecular marker club cell secretory protein (CCSP). The identity of alveolar stem cells remains incompletely understood. The ATII cell is generally believed to serve this function, although bronchiolar stem cell populations, which express α6β4 integrin, keratin 5, or p63 but not prosurfactant C, may function as alveolar epithelial progenitor cells after injury under certain circumstances.
Facultative Progenitor Cell Pools
A facultative progenitor cell is one that exhibits differentiated features in the quiescent state yet has the capacity to proliferate for maintenance of normal tissue and in response to injury. In contrast with the bone marrow and gut, the lung epithelium is maintained and repaired under most conditions by an abundant, broadly distributed facultative progenitor cell pool. Facultative progenitor cells exist in two states: quiescent and reparative. In the steady state, facultative progenitor cells are nonmitotic and carry out differentiated functions necessary for tissue homeostasis. In response to injury, facultative progenitor cells dedifferentiate, enter the cell cycle, self-renew, and have a context-dependent probability of differentiating into regionally specific differentiated cell types such as ciliated epithelial cells and ATII cells. Examples of facultative progenitor cells in the lung are the club cell in the airway and the ATII cell in the alveolus.
Loss of Regenerative Potential: Depletion of the Facultative Progenitor Cell Pool
Multifunctional properties of pulmonary facultative progenitor cells also limit their ability to repair the injured conducting airway epithelium in the mouse lung. First, the metabolic pathways that allow the club cell to eliminate lipophilic agents also sensitize it to the toxic effects of these compounds. Second, the phenotypic plasticity that is essential to the club cell’s ability to detect and eliminate pathogens may compromise the reparative functions of this cell type. Finally, repeated participation in epithelial repair may deplete the mitotic potential of the facultative progenitor cell pool. Depletion of this cell type would leave the epithelium deficient in both regenerative and differentiated functions and could result in a cascade of changes that contribute to epithelial fragility through loss of cellular autocrine/paracrine protective mechanisms, epithelial hypoplasia, and dysregulation of interactions between the epithelial, mesenchymal, and vascular compartments.
Histologic measures of a low abundance of club cells and CCSP support the concept that the facultative progenitor cell type(s) are depleted in acute lung injury and in chronic lung diseases. These studies suggest that four processes lead to depletion of this critical cell type. First, transition to a mucus-producing cell type may alter both progenitor function and cellular interactions. Within small airways of COPD patients and asthmatics, club cells undergo a metaplastic transition to a mucosecretory phenotype. In severe disease, both club cells and mucosecretory cell types are depleted, and the epithelium becomes hypoplastic. Analysis of colony-forming cells within the human bronchial epithelium demonstrated that human mucous cells are postmitotic cells. These observations suggest that hyperplasia of mucous cells, in the context of acute or chronic lung disease, is a consequence of a phenotypic transition by non–mucus-producing cells.
Second, transition of progenitor cells from a facultative to an obligate progenitor state may also lead to depletion of reparative cells. Increased epithelial proliferation in the lungs of smokers suggests an ongoing injury process and the potential for replicative senescence within the facultative progenitor cell pool. Senescent cells persist and consequently inhibit activation of the remaining reparative cells. Thus senescent cells could compromise differentiated functions while also blocking cell replacement mechanisms.
Third, entry of the facultative progenitor cell into the cell cycle is accompanied by loss of differentiated functions such as secretion of CCSP or production of surfactant. Extensive analysis of CCSP levels in acute and chronic lung disease, including COPD, suggests that differentiated functions of the facultative progenitor cells are compromised and that these cells are a direct target of toxic environmental agents. Thus injuries that activate facultative progenitor cells may adversely affect protective mechanisms and render the epithelium susceptible to further injury.
Finally, defects in cellular maturation may also contribute to a failure to establish the facultative progenitor cell pool or to deplete it after injury. The lung undergoes rapid and extensive maturation during the postnatal period. The maturation process is interrupted by preterm birth and associated oxygen treatment and is attenuated by postnatal exposure to environmental agents, including ozone and side-stream tobacco smoke in term infants. These studies support the concept that failure to establish or maintain an appropriately sized pool of facultative progenitor cells fosters environmentally induced chronic injury and dysfunctional repair cycles characteristic of lung disease. Hypoplasia of bronchial club cells is associated with tissue remodeling characterized by hyperplasia of an alternative progenitor, the basal cell, and consequent squamous metaplasia. In contrast, club and ATII cells are progenitors of the bronchiolar and alveolar epithelium, respectively, although as mentioned earlier, bronchiolar stem cells may repopulate the alveolus, and an alveolar epithelial cell that expresses α6β4 integrin, but not prosurfactant C, may function as an alveolar epithelial progenitor cell after injury. Depletion of either of these cell types leads to epithelial hypoplasia and remodeling of adjacent tissue compartments. Thus changes in function and/or abundance of the facultative progenitor may be the nexus for the complex pathophysiologic alterations in chronic lung diseases.
Gene-Environment Interactions in Lung Injury and Repair
The four Rs (regeneration, repair, remodeling, and replacement) are presented as distinct processes; however, they are actually intricately intertwined responses to acute or chronic lung injury ( Fig. 15-6 ). These relationships are modified by gene-environment interactions, interactions based on the concept that genetic constitution determines the response to deleterious agents within the environment.

Genetic predisposition has been associated with allelic variants that modify cellular susceptibility to environmental agents, including chemical (ozone, naphthalene, cigarette smoke) and microbial (lipopolysaccharides, viral pathogens) agents. Such alleles can sensitize an individual to an initial or repeated injury but can also render him or her resistant to subsequent exposures through induction of tolerance.
Genotype can also be a determinant of the cellular response to an agent. In mice, specific genetic backgrounds are susceptible to metaplastic transitions such as conversion of airway secretory cells into a mucus-producing goblet cell phenotype (e.g., C57BL/6 vs. Balb/c strains of mice). Such transitions are associated with protection of the epithelium from further injury. Alterations in cellular phenotype may also extend to interactions between endogenous lung elements (epithelium, mesenchyme, nerves) and itinerant (recruited) cells such as neutrophils, monocytes, and lymphocytes. Certain inbred strains of mice (e.g., C57BL/6) are more susceptible to bleomycin-induced inflammation and develop transient replacement of alveolar structures with mesenchymal cells resulting in fibrosis. Thus susceptibility to injury and the response to such an insult are determined by multiple factors, including the nature of the agent, dose and route of exposure, exposure history, the host genotype, and epigenetic factors molded by gene-environment interactions.
Does Lung Repair Recapitulate Lung Development?
Numerous signaling processes are known to be involved in lung development including Wnt/β-catenin, notch-delta, sonic hedgehog-patched, fibroblast growth factor–fibroblast growth factor receptor, and bone morphogenetic protein/TGF-β. These pathways are interactive and integrate formation and appropriate differentiation of tissues (epithelium, mesenchyme, vasculature) during lung development. During lung injury and repair, reactivation of many of these pathways has been reported. It is unclear, however, whether these various signaling pathways are integrated and whether this signaling results in productive cell replacement. Indeed, analysis of damaged human lung tissue is descriptive, and thus cause and consequence usually cannot be separated. In contrast, analysis of signaling pathways in animal models relies on elimination of a specific pathway or profound misregulation of the targeted process. Consequently, feedback mechanisms are likely to be disrupted and may result in altered activation/inactivation of parallel or sequential signaling networks. Development of methods to simultaneously monitor alterations in multiple signaling cascades is needed to address this fundamental obstacle to understanding the molecular regulation of reparative processes. Hence at this time it is not known if regulatory pathways that are dominant during development also regulate repair in the adult.
- ▪
The processes of lung injury and repair underpin the pathogenesis of myriad lung diseases, as well as the normal aging process.
- ▪
The mechanisms of injury are rooted in the cellular and molecular changes that initiate structural alterations and lead to compromised lung function. At the tissue and cellular level, exogenous insults and/or inflammatory stimuli result in increased vascular permeability and loss of alveolar epithelial barrier function due to disruption of intercellular junctions and cell death.
- ▪
Susceptibility to lung injury is determined by general health, previous exposures, and the individual’s genetic constitution. This “gene-environment interaction” is the cornerstone of research initiatives directed at identification of susceptibility genes and those that modify the response to treatment.
- ▪
Lung repair involves restoration of normal cellular composition, lung architecture, barrier function, and gas exchange.
- ▪
In response to cellular injury by noxious stimuli such as microbes and toxic compounds, endogenous stem and progenitor cells undergo rapid proliferation and differentiation resulting in repair and regeneration of the injured lung. Under normal circumstances repair of the alveolar epithelium after injury depends on cell spreading, migration, and proliferation of surviving alveolar epithelial type II (ATII) cells, followed by differentiation of ATII cells into ATI cells. However, in severe injury, additional non-surfactant protein C–expressing cells, likely from the bronchioalveolar junction, can repopulate the alveolar epithelium.
- ▪
Repetitive epithelial injury and dysfunctional repair can lead to fibroproliferative responses in the airways and parenchyma; fibroproliferative responses are thought to be critical to the pathogenesis of some diseases, including pulmonary fibrosis, COPD, and asthma.
- ▪
Ultimately, the inflammatory response must resolve in order to halt ongoing injury to the lung and to allow repair. Inflammatory cells undergo apoptosis and are cleared by macrophages in a phagocytic process termed “efferocytosis.”
Key Readings
References
- 1. Yoshida T, Mett I, Bhunia AK, et al: Rtp801, a suppressor of mTOR signaling, is an essential mediator of cigarette smoke-induced pulmonary injury and emphysema. Nat Med 2010; 16: pp. 767-773
- 2. Rangasamy T, Cho CY, Thimmulappa RK, et al: Genetic ablation of Nrf2 enhances susceptibility to cigarette smoke-induced emphysema in mice. J Clin Invest 2004; 114: pp. 1248-1259
- 3. Seimetz M, Parajuli N, Pichl A, et al: Inducible NOS inhibition reverses tobacco-smoke-induced emphysema and pulmonary hypertension in mice. Cell 2011; 147: pp. 293-305
- 4. Kasahara Y, Tuder RM, Taraseviciene-Stewart L, et al: Inhibition of VEGF receptors causes lung cell apoptosis and emphysema. J Clin Invest 2000; 106: pp. 1311-1319
- 5. Petrache I, Natarajan V, Zhen L, et al: Ceramide upregulation causes pulmonary cell apoptosis and emphysema-like disease in mice. Nat Med 2005; 11: pp. 491-498
- 6. Hautamaki RD, Kobayashi DK, Senior RM, et al: Requirement for macrophage elastase for cigarette smoke-induced emphysema in mice. Science 1997; 277: pp. 2002-2004
- 7. Davies DE: The role of the epithelium in airway remodeling in asthma. Proc Am Thorac Soc 2009; 6: pp. 678-682
- 8. Holgate ST, Roberts G, Arshad HS, et al: The role of the airway epithelium and its interaction with environmental factors in asthma pathogenesis. Proc Am Thorac Soc 2009; 6: pp. 655-659
- 9. Chanez P: Severe asthma is an epithelial disease. Eur Respir J 2005; 25: pp. 945-946
- 10. Chapman HA: Disorders of lung matrix remodeling. J Clin Invest 2004; 113: pp. 148-157
- 11. Zoz DF, Lawson WE, and Blackwell TS: Idiopathic pulmonary fibrosis: a disorder of epithelial cell dysfunction. Am J Med Sci 2011; 341: pp. 435-438
- 12. Ranieri VM, Rubenfeld GD, Thompson BT, et al: Acute respiratory distress syndrome: the Berlin definition. JAMA 2012; 307: pp. 2526-2533
- 13. Moss M, Bucher B, Moore FA, et al: The role of chronic alcohol abuse in the development of acute respiratory distress syndrome in adults. JAMA 1996; 275: pp. 50-54
- 14. Calfee CS, Matthay MA, Eisner MD, et al: Active and passive cigarette smoking and acute lung injury after severe blunt trauma. Am J Respir Crit Care Med 2011; 183: pp. 1660-1665
- 15. Marshall RP, Webb S, Bellingan GJ, et al: Angiotensin converting enzyme insertion/deletion polymorphism is associated with susceptibility and outcome in acute respiratory distress syndrome. Am J Respir Crit Care Med 2002; 166: pp. 646-650
- 16. Tejera P, Meyer NJ, Chen F, et al: Distinct and replicable genetic risk factors for acute respiratory distress syndrome of pulmonary or extrapulmonary origin. J Med Genet 2012; 49: pp. 671-680
- 17. O’Mahony DS, Glavan BJ, Holden TD, et al: Inflammation and immune-related candidate gene associations with acute lung injury susceptibility and severity: a validation study. PLoS ONE 2012; 7: pp. e51104
- 18. Christie JD, Wurfel MM, Feng R, et al: Genome wide association identifies PPFIA1 as a candidate gene for acute lung injury risk following major trauma. PLoS ONE 2012; 7: pp. e28268
- 19. Meyer NJ, Li M, Feng R, et al: ANGPT2 genetic variant is associated with trauma-associated acute lung injury and altered plasma angiopoietin-2 isoform ratio. Am J Respir Crit Care Med 2011; 183: pp. 1344-1353
- 20. Glavan BJ, Holden TD, Goss CH, et al: Genetic variation in the FAS gene and associations with acute lung injury. Am J Respir Crit Care Med 2011; 183: pp. 356-363
- 21. Marzec JM, Christie JD, Reddy SP, et al: Functional polymorphisms in the transcription factor NRF2 in humans increase the risk of acute lung injury. FASEB J 2007; 21: pp. 2237-2246
- 22. Ma SF, Xie L, Pino-Yanes M, et al: Type 2 deiodinase and host responses of sepsis and acute lung injury. Am J Respir Cell Mol Biol 2011; 45: pp. 1203-1211
- 23. Flores C, Ma SF, Maresso K, et al: IL6 gene-wide haplotype is associated with susceptibility to acute lung injury. Transl Res 2008; 152: pp. 11-17
- 24. Gao L, Flores C, Fan-Ma S, et al: Macrophage migration inhibitory factor in acute lung injury: expression, biomarker, and associations. Transl Res 2007; 150: pp. 18-29
- 25. Gao L, Grant A, Halder I, et al: Novel polymorphisms in the myosin light chain kinase gene confer risk for acute lung injury. Am J Respir Cell Mol Biol 2006; 34: pp. 487-495
- 26. Meyer NJ, Huang Y, Singleton PA, et al: GADD45a is a novel candidate gene in inflammatory lung injury via influences on Akt signaling. FASEB J 2009; 23: pp. 1325-1337
- 27. Ye SQ, Simon BA, Maloney JP, et al: Pre-B-cell colony-enhancing factor as a potential novel biomarker in acute lung injury. Am J Respir Crit Care Med 2005; 171: pp. 361-370
- 28. Garcia JG: Genomic investigations into acute inflammatory lung injury. Proc Am Thorac Soc 2011; 8: pp. 167-172
- 29. Grigoryev DN, Ma SF, Irizarry RA, et al: Orthologous gene-expression profiling in multi-species models: search for candidate genes. Genome Biol 2004; 5: pp. R34
- 30. Villar J, Flores C, and Mendez-Alvarez S: Genetic susceptibility to acute lung injury. Crit Care Med 2003; 31: pp. S272-S275
- 31. Leikauf GD, McDowell SA, Wesselkamper SC, et al: Acute lung injury: functional genomics and genetic susceptibility. Chest 2002; 121: pp. S70-S75
- 32. Pino-Yanes M, Ma SF, Sun X, et al: Interleukin-1 receptor-associated kinase 3 gene associates with susceptibility to acute lung injury. Am J Respir Cell Mol Biol 2011; 45: pp. 740-745
- 33. Gao L, and Barnes KC: Recent advances in genetic predisposition to clinical acute lung injury. Am J Physiol Lung Cell Mol Physiol 2009; 296: pp. L713-L725
- 34. Tejera P, Wang Z, Zhai R, et al: Genetic polymorphisms of peptidase inhibitor 3 (elafin) are associated with acute respiratory distress syndrome. Am J Respir Cell Mol Biol 2009; 41: pp. 696-704
- 35. Ventilation with lower tidal volumes as compared with traditional tidal volumes for acute lung injury and the acute respiratory distress syndrome. The Acute Respiratory Distress Syndrome Network. N Engl J Med 2000; 342: pp. 1301-1308
- 36. Wiedemann HP, Wheeler AP, Bernard GR, et al: Comparison of two fluid-management strategies in acute lung injury. N Engl J Med 2006; 354: pp. 2564-2575
- 37. Fein A, Grossman RF, Jones JG, et al: The value of edema fluid protein measurement in patients with pulmonary edema. Am J Med 1979; 67: pp. 32-38
- 38. Bachofen M, and Weibel ER: Structural alterations of lung parenchyma in the adult respiratory distress syndrome. Clin Chest Med 1982; 3: pp. 35-56
- 39. Matthay MA, Ware LB, and Zimmerman GA: The acute respiratory distress syndrome. J Clin Invest 2012; 122: pp. 2731-2740
- 40. Bachofen M, and Weibel ER: Alterations of the gas exchange apparatus in adult respiratory insufficiency associated with septicemia. Am Rev Respir Dis 1977; 116: pp. 589-615
- 41. Ware LB, and Matthay MA: The acute respiratory distress syndrome. N Engl J Med 2000; 342: pp. 1334-1349
- 42. Ashbaugh DG, Bigelow DB, Petty TL, et al: Acute respiratory distress in adults. Lancet 1967; 2: pp. 319-323
- 43. Ware LB, and Matthay MA: Alveolar fluid clearance is impaired in the majority of patients with acute lung injury and the acute respiratory distress syndrome. Am J Respir Crit Care Med 2001; 163: pp. 1376-1383
- 44. Dada LA, Chandel NS, Ridge KM, et al: Hypoxia-induced endocytosis of Na,K-ATPase in alveolar epithelial cells is mediated by mitochondrial reactive oxygen species and PKC-zeta. J Clin Invest 2003; 111: pp. 1057-1064
- 45. Gregory TJ, Longmore WJ, Moxley MA, et al: Surfactant chemical composition and biophysical activity in acute respiratory distress syndrome. J Clin Invest 1991; 88: pp. 1976-1981
- 46. Albert RK, Lakshminarayan S, Hildebrandt J, et al: Increased surface tension favors pulmonary edema formation in anesthetized dogs’ lungs. J Clin Invest 1979; 63: pp. 1015-1018
- 47. Rinaldo JE: Mediation of ARDS by leukocytes. Clinical evidence and implications for therapy. Chest 1986; 89: pp. 590-593
- 48. Mizgerd JP: Acute lower respiratory tract infection. N Engl J Med 2008; 358: pp. 716-727
- 49. Hammerschmidt DE, Weaver LJ, Hudson LD, et al: Association of complement activation and elevated plasma-C5a with adult respiratory distress syndrome. Pathophysiological relevance and possible prognostic value. Lancet 1980; 1: pp. 947-949
- 50. Abraham E, Carmody A, Shenkar R, et al: Neutrophils as early immunologic effectors in hemorrhage- or endotoxemia-induced acute lung injury. Am J Physiol Lung Cell Mol Physiol 2000; 279: pp. L1137-L1145
- 51. Standiford TJ, Kunkel SL, Basha MA, et al: Interleukin-8 gene expression by a pulmonary epithelial cell line. A model for cytokine networks in the lung. J Clin Invest 1990; 86: pp. 1945-1953
- 52. Steinberg KP, Milberg JA, Martin TR, et al: Evolution of bronchoalveolar cell populations in the adult respiratory distress syndrome. Am J Respir Crit Care Med 1994; 150: pp. 113-122
- 53. Worthen GS, Schwab B, Elson EL, et al: Mechanics of stimulated neutrophils: cell stiffening induces retention in capillaries. Science 1989; 245: pp. 183-186
- 54. Doyle NA, Bhagwan SD, Meek BB, et al: Neutrophil margination, sequestration, and emigration in the lungs of L-selectin-deficient mice. J Clin Invest 1997; 99: pp. 526-533
- 55. Doerschuk CM, Winn RK, Coxson HO, et al: CD18-dependent and -independent mechanisms of neutrophil emigration in the pulmonary and systemic microcirculation of rabbits. J Immunol 1990; 144: pp. 2327-2333
- 56. Zimmerman GA, McIntyre TM, and Prescott SM: Thrombin stimulates the adherence of neutrophils to human endothelial cells in vitro. J Clin Invest 1985; 76: pp. 2235-2246
- 57. Schmidt EP, Yang Y, Janssen WJ, et al: The pulmonary endothelial glycocalyx regulates neutrophil adhesion and lung injury during experimental sepsis. Nat Med 2012; 18: pp. 1217-1223
- 58. Thommasen HV, Russell JA, Boyko WJ, et al: Transient leucopenia associated with adult respiratory distress syndrome. Lancet 1984; 1: pp. 809-812
- 59. Zemans RL, Colgan SP, and Downey GP: Transepithelial migration of neutrophils: mechanisms and implications for acute lung injury. Am J Respir Cell Mol Biol 2009; 40: pp. 519-535
- 60. Wang Q, Doerschuk CM, and Mizgerd JP: Neutrophils in innate immunity. Semin Respir Crit Care Med 2004; 25: pp. 33-41
- 61. Martin TR: Neutrophils and lung injury: getting it right. J Clin Invest 2002; 110: pp. 1603-1605
- 62. Martin TR, Pistorese BP, Chi EY, et al: Effects of leukotriene B4 in the human lung. Recruitment of neutrophils into the alveolar spaces without a change in protein permeability. J Clin Invest 1989; 84: pp. 1609-1619
- 63. Lee WL, and Downey GP: Neutrophil activation and acute lung injury. Curr Opin Crit Care 2001; 7: pp. 1-7
- 64. Diep BA, Chan L, Tattevin P, et al: Polymorphonuclear leukocytes mediate . Proc Natl Acad Sci U S A 2010; 107: pp. 5587-5592
- 65. Belaaouaj A, Kim KS, and Shapiro SD: Degradation of outer membrane protein A in . Science 2000; 289: pp. 1185-1188
- 66. Belaaouaj A, McCarthy R, Baumann M, et al: Mice lacking neutrophil elastase reveal impaired host defense against gram negative bacterial sepsis. Nat Med 1998; 4: pp. 615-618
- 67. Stoller JK, and Aboussouan LS: Alpha1-antitrypsin deficiency. Lancet 2005; 365: pp. 2225-2236
- 68. Shapiro SD: Neutrophil elastase: path clearer, pathogen killer, or just pathologic? Am J Respir Cell Mol Biol 2002; 26: pp. 266-268
- 69. Lee WL, and Downey GP: Leukocyte elastase: physiological functions and role in acute lung injury. Am J Respir Crit Care Med 2001; 164: pp. 896-904
- 70. Delacourt C, Herigault S, Delclaux C, et al: Protection against acute lung injury by intravenous or intratracheal pretreatment with EPI-HNE-4, a new potent neutrophil elastase inhibitor. Am J Respir Cell Mol Biol 2002; 26: pp. 290-297
- 71. Gossage JR, Kuratomi Y, Davidson JM, et al: Neutrophil elastase inhibitors, SC-37698 and SC-39026, reduce endotoxin-induced lung dysfunction in awake sheep. Am Rev Respir Dis 1993; 147: pp. 1371-1379
- 72. Sakamaki F, Ishizaka A, Urano T, et al: Effect of a specific neutrophil elastase inhibitor, ONO-5046, on endotoxin-induced acute lung injury. Am J Respir Crit Care Med 1996; 153: pp. 391-397
- 73. Kawabata K, Hagio T, Matsumoto S, et al: Delayed neutrophil elastase inhibition prevents subsequent progression of acute lung injury induced by endotoxin inhalation in hamsters. Am J Respir Crit Care Med 2000; 161: pp. 2013-2018
- 74. Takayama M, Ishibashi M, Ishii H, et al: Effects of neutrophil elastase inhibitor (ONO-5046) on lung injury after intestinal ischemia-reperfusion. J Appl Physiol 2001; 91: pp. 1800-1807
- 75. Tkalcevic J, Novelli M, Phylactides M, et al: Impaired immunity and enhanced resistance to endotoxin in the absence of neutrophil elastase and cathepsin G. Immunity 2000; 12: pp. 201-210
- 76. Hilgendorff A, Parai K, Ertsey R, et al: Inhibiting lung elastase activity enables lung growth in mechanically ventilated newborn mice. Am J Respir Crit Care Med 2011; 184: pp. 537-546
- 77. Ginzberg HH, Cherapanov V, Dong Q, et al: Neutrophil-mediated epithelial injury during transmigration: role of elastase. Am J Physiol Gastrointest Liver Physiol 2001; 281: pp. G705-G717
- 78. Carden D, Xiao F, Moak C, et al: Neutrophil elastase promotes lung microvascular injury and proteolysis of endothelial cadherins. Am J Physiol 1998; 275: pp. H385-H392
- 79. Smedly LA, Tonnesen MG, Sandhaus RA, et al: Neutrophil-mediated injury to endothelial cells. Enhancement by endotoxin and essential role of neutrophil elastase. J Clin Invest 1986; 77: pp. 1233-1243
- 80. Harlan JM, Killen PD, Harker LA, et al: Neutrophil-mediated endothelial injury in vitro mechanisms of cell detachment. J Clin Invest 1981; 68: pp. 1394-1403
- 81. Yang JJ, Kettritz R, Falk RJ, et al: Apoptosis of endothelial cells induced by the neutrophil serine proteases proteinase 3 and elastase. Am J Pathol 1996; 149: pp. 1617-1626
- 82. Ginzberg HH, Shannon PT, Suzuki T, et al: Leukocyte elastase induces epithelial apoptosis: role of mitochondial permeability changes and Akt. Am J Physiol Gastrointest Liver Physiol 2004; 287: pp. G286-G298
- 83. Suzuki T, Moraes TJ, Vachon E, et al: Proteinase-activated receptor-1 mediates elastase-induced apoptosis of human lung epithelial cells. Am J Respir Cell Mol Biol 2005; 33: pp. 231-247
- 84. Lee CT, Fein AM, Lippmann M, et al: Elastolytic activity in pulmonary lavage fluid from patients with adult respiratory-distress syndrome. N Engl J Med 1981; 304: pp. 192-196
- 85. Idell S, Kucich U, Fein A, et al: Neutrophil elastase-releasing factors in bronchoalveolar lavage from patients with adult respiratory distress syndrome. Am Rev Respir Dis 1985; 132: pp. 1098-1105
- 86. Cochrane CG, Spragg RG, Revak SD, et al: The presence of neutrophil elastase and evidence of oxidation activity in bronchoalveolar lavage fluid of patients with adult respiratory distress syndrome. Am Rev Respir Dis 1983; 127: pp. S25-S27
- 87. Donnelly SC, MacGregor I, Zamani A, et al: Plasma elastase levels and the development of the adult respiratory distress syndrome. Am J Respir Crit Care Med 1995; 151: pp. 1428-1433
- 88. Zeiher BG, Artigas A, Vincent JL, et al: Neutrophil elastase inhibition in acute lung injury: results of the STRIVE study. Crit Care Med 2004; 32: pp. 1695-1702
- 89. Iwata K, Doi A, Ohji G, et al: Effect of neutrophil elastase inhibitor (sivelestat sodium) in the treatment of acute lung injury (ALI) and acute respiratory distress syndrome (ARDS): a systematic review and meta-analysis. Intern Med 2010; 49: pp. 2423-2432
- 90. Torii K, Iida K, Miyazaki Y, et al: Higher concentrations of matrix metalloproteinases in bronchoalveolar lavage fluid of patients with adult respiratory distress syndrome. Am J Respir Crit Care Med 1997; 155: pp. 43-46
- 91. Ricou B, Nicod L, Lacraz S, et al: Matrix metalloproteinases and TIMP in acute respiratory distress syndrome. Am J Respir Crit Care Med 1996; 154: pp. 346-352
- 92. Delclaux C, Delacourt C, D’Ortho MP, et al: Role of gelatinase B and elastase in human polymorphonuclear neutrophil migration across basement membrane. Am J Respir Cell Mol Biol 1996; 14: pp. 288-295
- 93. Fligiel SE, Standiford T, Fligiel HM, et al: Matrix metalloproteinases and matrix metalloproteinase inhibitors in acute lung injury. Hum Pathol 2006; 37: pp. 422-430
- 94. Pugin J, Ricou B, Steinberg KP, et al: Proinflammatory activity in bronchoalveolar lavage fluids from patients with ARDS, a prominent role for interleukin-1. Am J Respir Crit Care Med 1996; 153: pp. 1850-1856
- 95. Carney DE, Lutz CJ, Picone AL, et al: Matrix metalloproteinase inhibitor prevents acute lung injury after cardiopulmonary bypass. Circulation 1999; 100: pp. 400-406
- 96. Kim JH, Suk MH, Yoon DW, et al: Inhibition of matrix metalloproteinase-9 prevents neutrophilic inflammation in ventilator-induced lung injury. Am J Physiol Lung Cell Mol Physiol 2006; 291: pp. L580-L587
- 97. Kim KH, Burkhart K, Chen P, et al: Tissue inhibitor of metalloproteinase-1 deficiency amplifies acute lung injury in bleomycin-exposed mice. Am J Respir Cell Mol Biol 2005; 33: pp. 271-279
- 98. Warner RL, Beltran L, Younkin EM, et al: Role of stromelysin 1 and gelatinase B in experimental acute lung injury. Am J Respir Cell Mol Biol 2001; 24: pp. 537-544
- 99. Warner RL, Lewis CS, Beltran L, et al: The role of metalloelastase in immune complex-induced acute lung injury. Am J Pathol 2001; 158: pp. 2139-2144
- 100. Pardo A, Selman M, Ridge K, et al: Increased expression of gelatinases and collagenase in rat lungs exposed to 100% oxygen. Am J Respir Crit Care Med 1996; 154: pp. 1067-1075
- 101. Gorodeski GI: Estrogen decrease in tight junctional resistance involves matrix-metalloproteinase-7-mediated remodeling of occludin. Endocrinology 2007; 148: pp. 218-231
- 102. Pflugfelder SC, Farley W, Luo L, et al: Matrix metalloproteinase-9 knockout confers resistance to corneal epithelial barrier disruption in experimental dry eye. Am J Pathol 2005; 166: pp. 61-71
- 103. Vermeer PD, Denker J, Estin M, et al: MMP9 modulates tight junction integrity and cell viability in human airway epithelia. Am J Physiol Lung Cell Mol Physiol 2009; 296: pp. L751-L762
- 104. Alexander JS, and Elrod JW: Extracellular matrix, junctional integrity and matrix metalloproteinase interactions in endothelial permeability regulation. J Anat 2002; 200: pp. 561-574
- 105. Navaratna D, McGuire PG, Menicucci G, et al: Proteolytic degradation of VE-cadherin alters the blood-retinal barrier in diabetes. Diabetes 2007; 56: pp. 2380-2387
- 106. Manicone AM, Harju-Baker S, Johnston LK, et al: Epilysin (matrix metalloproteinase-28) contributes to airway epithelial cell survival. Respir Res 2011; 12: pp. 144
- 107. Quintero PA, Knolle MD, Cala LF, et al: Matrix metalloproteinase-8 inactivates macrophage inflammatory protein-1 alpha to reduce acute lung inflammation and injury in mice. J Immunol 2010; 184: pp. 1575-1588
- 108. Gonzalez-Lopez A, Astudillo A, Garcia-Prieto E, et al: Inflammation and matrix remodeling during repair of ventilator-induced lung injury. Am J Physiol Lung Cell Mol Physiol 2011; 301: pp. L500-L509
- 109. Davey A, McAuley DF, and O’Kane CM: Matrix metalloproteinases in acute lung injury: mediators of injury and drivers of repair. Eur Respir J 2011; 38: pp. 959-970
- 110. Ashitani J, Mukae H, Arimura Y, et al: High concentrations of alpha-defensins in plasma and bronchoalveolar lavage fluid of patients with acute respiratory distress syndrome. Life Sci 2004; 75: pp. 1123-1134
- 111. Zhang H, Porro G, Orzech N, et al: Neutrophil defensins mediate acute inflammatory response and lung dysfunction in dose-related fashion. Am J Physiol Lung Cell Mol Physiol 2001; 280: pp. L947-L954
- 112. Bdeir K, Higazi AA, Kulikovskaya I, et al: Neutrophil alpha-defensins cause lung injury by disrupting the capillary-epithelial barrier. Am J Respir Crit Care Med 2010; 181: pp. 935-946
- 113. Okrent DG, Lichtenstein AK, and Ganz T: Direct cytotoxicity of polymorphonuclear leukocyte granule proteins to human lung-derived cells and endothelial cells. Am Rev Respir Dis 1990; 141: pp. 179-185
- 114. Aarbiou J, Tjabringa GS, Verhoosel RM, et al: Mechanisms of cell death induced by the neutrophil antimicrobial peptides alpha-defensins and LL-37. Inflamm Res 2006; 55: pp. 119-127
- 115. Van Wetering S, Mannesse-Lazeroms SP, Dijkman JH, et al: Effect of neutrophil serine proteinases and defensins on lung epithelial cells: modulation of cytotoxicity and IL-8 production. J Leukoc Biol 1997; 62: pp. 217-226
- 116. Sakamoto N, Mukae H, Fujii T, et al: Differential effects of alpha- and beta-defensin on cytokine production by cultured human bronchial epithelial cells. Am J Physiol Lung Cell Mol Physiol 2005; 288: pp. L508-L513
- 117. Saito A, Ariki S, Sohma H, et al: Pulmonary surfactant protein A protects lung epithelium from cytotoxicity of human beta-defensin 3. J Biol Chem 2012; 287: pp. 15034-15043
- 118. Nygaard SD, Ganz T, and Peterson MW: Defensins reduce the barrier integrity of a cultured epithelial monolayer without cytotoxicity. Am J Respir Cell Mol Biol 1993; 8: pp. 193-200
- 119. Aarbiou J, Verhoosel RM, Van Wetering S, et al: Neutrophil defensins enhance lung epithelial wound closure and mucin gene expression in vitro. Am J Respir Cell Mol Biol 2004; 30: pp. 193-201
- 120. Aarbiou J, Ertmann M, van Wetering S, et al: Human neutrophil defensins induce lung epithelial cell proliferation in vitro. J Leukoc Biol 2002; 72: pp. 167-174
- 121. Sznajder JI, Fraiman A, Hall JB, et al: Increased hydrogen peroxide in the expired breath of patients with acute hypoxemic respiratory failure. Chest 1989; 96: pp. 606-612
- 122. Sittipunt C, Steinberg KP, Ruzinski JT, et al: Nitric oxide and nitrotyrosine in the lungs of patients with acute respiratory distress syndrome. Am J Respir Crit Care Med 2001; 163: pp. 503-510
- 123. Zhu S, Ware LB, Geiser T, et al: Increased levels of nitrate and surfactant protein a nitration in the pulmonary edema fluid of patients with acute lung injury. Am J Respir Crit Care Med 2001; 163: pp. 166-172
- 124. Fink MP: Role of reactive oxygen and nitrogen species in acute respiratory distress syndrome. Curr Opin Crit Care 2002; 8: pp. 6-11
- 125. Kristof AS, Goldberg P, Laubach V, et al: Role of inducible nitric oxide synthase in endotoxin-induced acute lung injury. Am J Respir Crit Care Med 1998; 158: pp. 1883-1889
- 126. Wang W, Suzuki Y, Tanigaki T, et al: Effect of the NADPH oxidase inhibitor apocynin on septic lung injury in guinea pigs. Am J Respir Crit Care Med 1994; 150: pp. 1449-1452
- 127. Lang JD, McArdle PJ, O’Reilly PJ, et al: Oxidant-antioxidant balance in acute lung injury. Chest 2002; 122: pp. S314-S320
- 128. Johnson KJ, Fantone JC, Kaplan J, et al: In vivo damage of rat lungs by oxygen metabolites. J Clin Invest 1981; 67: pp. 983-993
- 129. Berisha HI, Pakbaz H, Absood A, et al: Nitric oxide as a mediator of oxidant lung injury due to paraquat. Proc Natl Acad Sci U S A 1994; 91: pp. 7445-7449
- 130. Li Z, Potts EN, Piantadosi CA, et al: Hyaluronan fragments contribute to the ozone-primed immune response to lipopolysaccharide. J Immunol 2010; 185: pp. 6891-6898
- 131. Ward PA: Oxidative stress: acute and progressive lung injury. Ann N Y Acad Sci 2010; 1203: pp. 53-59
- 132. Imai Y, Kuba K, Neely GG, et al: Identification of oxidative stress and Toll-like receptor 4 signaling as a key pathway of acute lung injury. Cell 2008; 133: pp. 235-249
- 133. Budinger GR, Mutlu GM, Urich D, et al: Epithelial cell death is an important contributor to oxidant-mediated acute lung injury. Am J Respir Crit Care Med 2011; 183: pp. 1043-1054
- 134. Barazzone C, Horowitz S, Donati YR, et al: Oxygen toxicity in mouse lung: pathways to cell death. Am J Respir Cell Mol Biol 1998; 19: pp. 573-581
- 135. Buckley S, Barsky L, Driscoll B, et al: Apoptosis and DNA damage in type 2 alveolar epithelial cells cultured from hyperoxic rats. Am J Physiol 1998; 274: pp. L714-L720
- 136. De Paepe ME, Mao Q, Chao Y, et al: Hyperoxia-induced apoptosis and Fas/FasL expression in lung epithelial cells. Am J Physiol Lung Cell Mol Physiol 2005; 289: pp. L647-L659
- 137. Jyonouchi H, Sun S, Abiru T, et al: The effects of hyperoxic injury and antioxidant vitamins on death and proliferation of human small airway epithelial cells. Am J Respir Cell Mol Biol 1998; 19: pp. 426-436
- 138. Kazzaz JA, Xu J, Palaia TA, et al: Cellular oxygen toxicity. Oxidant injury without apoptosis. J Biol Chem 1996; 271: pp. 15182-15186
- 139. Otterbein LE, Chin BY, Mantell LL, et al: Pulmonary apoptosis in aged and oxygen-tolerant rats exposed to hyperoxia. Am J Physiol 1998; 275: pp. L14-L20
- 140. Pagano A, and Barazzone-Argiroffo C: Alveolar cell death in hyperoxia-induced lung injury. Ann N Y Acad Sci 2003; 1010: pp. 405-416
- 141. Zhang X, Shan P, Sasidhar M, et al: Reactive oxygen species and extracellular signal-regulated kinase 1/2 mitogen-activated protein kinase mediate hyperoxia-induced cell death in lung epithelium. Am J Respir Cell Mol Biol 2003; 28: pp. 305-315
- 142. Sacks T, Moldow CF, Craddock PR, et al: Oxygen radicals mediate endothelial cell damage by complement-stimulated granulocytes. An in vitro model of immune vascular damage. J Clin Invest 1978; 61: pp. 1161-1167
- 143. Varani J, Fligiel SE, Till GO, et al: Pulmonary endothelial cell killing by human neutrophils. Possible involvement of hydroxyl radical. Lab Invest 1985; 53: pp. 656-663
- 144. Kevil CG, Oshima T, Alexander B, et al: H(2)O(2)-mediated permeability: role of MAPK and occludin. Am J Physiol Cell Physiol 2000; 279: pp. C21-C30
- 145. Cheek JM, Postlethwait EM, Shaw ME, et al: Effects of exposure to NO2 on dome formation in alveolar epithelial cell monolayers. Environ Res 1987; 42: pp. 1-11
- 146. Chapman KE, Waters CM, and Miller WM: Continuous exposure of airway epithelial cells to hydrogen peroxide: protection by KGF. J Cell Physiol 2002; 192: pp. 71-80
- 147. Folz RJ, Abushamaa AM, and Suliman HB: Extracellular superoxide dismutase in the airways of transgenic mice reduces inflammation and attenuates lung toxicity following hyperoxia. J Clin Invest 1999; 103: pp. 1055-1066
- 148. Bowler RP, Arcaroli J, Abraham E, et al: Evidence for extracellular superoxide dismutase as a mediator of hemorrhage-induced lung injury. Am J Physiol Lung Cell Mol Physiol 2003; 284: pp. L680-L687
- 149. Kosmider B, Messier EM, Janssen WJ, et al: Nrf2 protects human alveolar epithelial cells against injury induced by influenza A virus. Respir Res 2012; 13: pp. 43
- 150. Reddy NM, Potteti HR, Mariani TJ, et al: Conditional deletion of Nrf2 in airway epithelium exacerbates acute lung injury and impairs the resolution of inflammation. Am J Respir Cell Mol Biol 2011; 45: pp. 1161-1168
- 151. Raval CM, and Lee PJ: Heme oxygenase-1 in lung disease. Curr Drug Targets 2010; 11: pp. 1532-1540
- 152. Lee PJ, Alam J, Wiegand GW, et al: Overexpression of heme oxygenase-1 in human pulmonary epithelial cells results in cell growth arrest and increased resistance to hyperoxia. Proc Natl Acad Sci U S A 1996; 93: pp. 10393-10398
- 153. Cho HY, Gladwell W, Wang X, et al: Nrf2-regulated PPARγ expression is critical to protection against acute lung injury in mice. Am J Respir Crit Care Med 2010; 182: pp. 170-182
- 154. Sohn MH, Kang MJ, Matsuura H, et al: The chitinase-like proteins breast regression protein-39 and YKL-40 regulate hyperoxia-induced acute lung injury. Am J Respir Crit Care Med 2010; 182: pp. 918-928
- 155. Ballinger MN, Newstead MW, Zeng X, et al: TLR signaling prevents hyperoxia-induced lung injury by protecting the alveolar epithelium from oxidant-mediated death. J Immunol 2012; 189: pp. 356-364
- 156. Baird BR, Cheronis JC, Sandhaus RA, et al: O2 metabolites and neutrophil elastase synergistically cause edematous injury in isolated rat lungs. J Appl Physiol 1986; 61: pp. 2224-2229
- 157. Saffarzadeh M, Juenemann C, Queisser MA, et al: Neutrophil extracellular traps directly induce epithelial and endothelial cell death: a predominant role of histones. PLoS ONE 2012; 7: pp. e32366
- 158. Caudrillier A, Kessenbrock K, Gilliss BM, et al: Platelets induce neutrophil extracellular traps in transfusion-related acute lung injury. J Clin Invest 2012; 122: pp. 2661-2671
- 159. Abrams ST, Zhang N, Manson J, et al: Circulating histones are mediators of trauma-associated lung injury. Am J Respir Crit Care Med 2013; 187: pp. 160-169
- 160. Xu J, Zhang X, Pelayo R, et al: Extracellular histones are major mediators of death in sepsis. Nat Med 2009; 15: pp. 1318-1321
- 161. Folkesson HG, Matthay MA, Hebert CA, et al: Acid aspiration-induced lung injury in rabbits is mediated by interleukin-8-dependent mechanisms. J Clin Invest 1995; 96: pp. 107-116
- 162. Grommes J, and Soehnlein O: Contribution of neutrophils to acute lung injury. Mol Med 2011; 17: pp. 293-307
- 163. Belperio JA, Keane MP, Burdick MD, et al: Critical role for CXCR2 and CXCR2 ligands during the pathogenesis of ventilator-induced lung injury. J Clin Invest 2002; 110: pp. 1703-1716
- 164. Ognibene FP, Martin SE, Parker MM, et al: Adult respiratory distress syndrome in patients with severe neutropenia. N Engl J Med 1986; 315: pp. 547-551
- 165. Rosseau S, Hammerl P, Maus U, et al: Phenotypic characterization of alveolar monocyte recruitment in acute respiratory distress syndrome. Am J Physiol Lung Cell Mol Physiol 2000; 279: pp. L25-L35
- 165a. Westphalen K, Gusarova GA, Islam MN, et al: Sessile alveolar macrophages communicate with alveolar epithelium to modulate immunity. Nature 2014; 506: pp. 503-506
- 166. Gossart S, Cambon C, Orfila C, et al: Reactive oxygen intermediates as regulators of TNF-alpha production in rat lung inflammation induced by silica. J Immunol 1996; 156: pp. 1540-1548
- 167. Haddad IY, Pataki G, Hu P, et al: Quantitation of nitrotyrosine levels in lung sections of patients and animals with acute lung injury. J Clin Invest 1994; 94: pp. 2407-2413
- 168. Gibbs DF, Shanley TP, Warner RL, et al: Role of matrix metalloproteinases in models of macrophage-dependent acute lung injury. Evidence for alveolar macrophage as source of proteinases. Am J Respir Cell Mol Biol 1999; 20: pp. 1145-1154
- 169. Herold S, Steinmueller M, von Wulffen W, et al: Lung epithelial apoptosis in influenza virus pneumonia: the role of macrophage-expressed TNF-related apoptosis-inducing ligand. J Exp Med 2008; 205: pp. 3065-3077
- 170. Thickett DR, Armstrong L, Christie SJ, et al: Vascular endothelial growth factor may contribute to increased vascular permeability in acute respiratory distress syndrome. Am J Respir Crit Care Med 2001; 164: pp. 1601-1605
- 171. Hogner K, Wolff T, Pleschka S, et al: Macrophage-expressed IFN-beta contributes to apoptotic alveolar epithelial cell injury in severe influenza virus pneumonia. PLoS Pathog 2013; 9: pp. e1003188
- 172. Frank JA, Wray CM, McAuley DF, et al: Alveolar macrophages contribute to alveolar barrier dysfunction in ventilator-induced lung injury. Am J Physiol Lung Cell Mol Physiol 2006; 291: pp. L1191-L1198
- 173. Wilson MR, O’Dea KP, Zhang D, et al: Role of lung-marginated monocytes in an in vivo mouse model of ventilator-induced lung injury. Am J Respir Crit Care Med 2009; 179: pp. 914-922
- 173a. Aggarwal NR, King LS, and D’Alessio FR: Diverse macrophage populations mediate acute lung inflammation and resolution. Am J Physiol Lung Cell Mol Physiol 2014; 306: pp. L709-L725
- 174. McGuire JK, Li Q, and Parks WC: Matrilysin (matrix metalloproteinase-7) mediates E-cadherin ectodomain shedding in injured lung epithelium. Am J Pathol 2003; 162: pp. 1831-1843
- 175. Gill SE, and Parks WC: Metalloproteinases and their inhibitors: regulators of wound healing. Int J Biochem Cell Biol 2008; 40: pp. 1334-1347
- 176. Novak ML, and Koh TJ: Macrophage phenotypes during tissue repair. J Leukoc Biol 2013; 93: pp. 875-881
- 177. Savill JS, Wyllie AH, Henson JE, et al: Macrophage phagocytosis of aging neutrophils in inflammation. Programmed cell death in the neutrophil leads to its recognition by macrophages. J Clin Invest 1989; 83: pp. 865-875
- 178. deCathelineau AM, and Henson PM: The final step in programmed cell death: phagocytes carry apoptotic cells to the grave. Essays Biochem 2003; 39: pp. 105-117
- 179. Looney MR, Nguyen JX, Hu Y, et al: Platelet depletion and aspirin treatment protect mice in a two-event model of transfusion-related acute lung injury. J Clin Invest 2009; 119: pp. 3450-3461
- 180. Bastarache JA, Fremont RD, Kropski JA, et al: Procoagulant alveolar microparticles in the lungs of patients with acute respiratory distress syndrome. Am J Physiol Lung Cell Mol Physiol 2009; 297: pp. L1035-L1041
- 181. Lucas R, Sridhar S, Rick FG, et al: Agonist of growth hormone-releasing hormone reduces pneumolysin-induced pulmonary permeability edema. Proc Natl Acad Sci U S A 2012; 109: pp. 2084-2089
- 182. White CW, and Martin JG: Chlorine gas inhalation: human clinical evidence of toxicity and experience in animal models. Proc Am Thorac Soc 2010; 7: pp. 257-263
- 183. Aggarwal NR, D’Alessio FR, Tsushima K, et al: Moderate oxygen augments lipopolysaccharide-induced lung injury in mice. Am J Physiol Lung Cell Mol Physiol 2010; 298: pp. L371-L381
- 184. Kuebler WM, Ying X, Singh B, et al: Pressure is proinflammatory in lung venular capillaries. J Clin Invest 1999; 104: pp. 495-502
- 185. Frank JA, Gutierrez JA, Jones KD, et al: Low tidal volume reduces epithelial and endothelial injury in acid-injured rat lungs. Am J Respir Crit Care Med 2002; 165: pp. 242-249
- 186. Imai Y, Kuba K, Rao S, et al: Angiotensin-converting enzyme 2 protects from severe acute lung failure. Nature 2005; 436: pp. 112-116
- 187. Dolinay T, Kim YS, Howrylak J, et al: Inflammasome-regulated cytokines are critical mediators of acute lung injury. Am J Respir Crit Care Med 2012; 185: pp. 1225-1234
- 188. Parthasarathi K, Ichimura H, Monma E, et al: Connexin 43 mediates spread of Ca2+-dependent proinflammatory responses in lung capillaries. J Clin Invest 2006; 116: pp. 2193-2200
- 189. Donnelly SC, Haslett C, Reid PT, et al: Regulatory role for macrophage migration inhibitory factor in acute respiratory distress syndrome. Nat Med 1997; 3: pp. 320-323
- 190. Zhang Q, Raoof M, Chen Y, et al: Circulating mitochondrial DAMPs cause inflammatory responses to injury. Nature 2010; 464: pp. 104-107
- 191. Jiang D, Liang J, Fan J, et al: Regulation of lung injury and repair by Toll-like receptors and hyaluronan. Nat Med 2005; 11: pp. 1173-1179
- 192. Pittet JF, Griffiths MJ, Geiser T, et al: TGF-beta is a critical mediator of acute lung injury. J Clin Invest 2001; 107: pp. 1537-1544
- 193. Su G, Hodnett M, Wu N, et al: Integrin alphavbeta5 regulates lung vascular permeability and pulmonary endothelial barrier function. Am J Respir Cell Mol Biol 2007; 36: pp. 377-386
- 194. Finigan JH, Faress JA, Wilkinson E, et al: Neuregulin-1-human epidermal receptor-2 signaling is a central regulator of pulmonary epithelial permeability and acute lung injury. J Biol Chem 2011; 286: pp. 10660-10670
- 195. Baker CS, Evans TW, Randle BJ, et al: Damage to surfactant-specific protein in acute respiratory distress syndrome. Lancet 1999; 353: pp. 1232-1237
- 196. Wright JR: Immunoregulatory functions of surfactant proteins. Nat Rev Immunol 2005; 5: pp. 58-68
- 197. Schulte D, Kuppers V, Dartsch N, et al: Stabilizing the VE-cadherin-catenin complex blocks leukocyte extravasation and vascular permeability. EMBO J 2011; 30: pp. 4157-4170
- 198. Broermann A, Winderlich M, Block H, et al: Dissociation of VE-PTP from VE-cadherin is required for leukocyte extravasation and for VEGF-induced vascular permeability in vivo. J Exp Med 2011; 208: pp. 2393-2401
- 199. Gao X, Kouklis P, Xu N, et al: Reversibility of increased microvessel permeability in response to VE-cadherin disassembly. Am J Physiol Lung Cell Mol Physiol 2000; 279: pp. L1218-L1225
- 200. Corada M, Mariotti M, Thurston G, et al: Vascular endothelial-cadherin is an important determinant of microvascular integrity in vivo. Proc Natl Acad Sci U S A 1999; 96: pp. 9815-9820
- 201. Hurst VI, Goldberg PL, Minnear FL, et al: Rearrangement of adherens junctions by transforming growth factor-beta1: role of contraction. Am J Physiol 1999; 276: pp. L582-L595
- 202. Ware LB, and Matthay MA: Maximal alveolar epithelial fliud clearance in clinical acute lung injury: an excellent predictor of survival and the duration of mechanical ventilation. Am J Respir Crit Care Med 1999; 159: pp. A694
- 203. Pittet JF, Lu LN, Morris DG, et al: Reactive nitrogen species inhibit alveolar epithelial fluid transport after hemorrhagic shock in rats. J Immunol 2001; 166: pp. 6301-6310
- 204. Lee JW, Fang X, Dolganov G, et al: Acute lung injury edema fluid decreases net fluid transport across human alveolar epithelial type II cells. J Biol Chem 2007; 282: pp. 24109-24119
- 205. Factor P, Saldias F, Ridge K, et al: Augmentation of lung liquid clearance via adenovirus-mediated transfer of a Na,K-ATPase beta1 subunit gene. J Clin Invest 1998; 102: pp. 1421-1430
- 206. Adir Y, Welch LC, Dumasius V, et al: Overexpression of the Na-K-ATPase alpha2-subunit improves lung liquid clearance during ventilation-induced lung injury. Am J Physiol Lung Cell Mol Physiol 2008; 294: pp. L1233-L1237
- 207. Litvan J, Briva A, Wilson MS, et al: Beta-adrenergic receptor stimulation and adenoviral overexpression of superoxide dismutase prevent the hypoxia-mediated decrease in Na,K-ATPase and alveolar fluid reabsorption. J Biol Chem 2006; 281: pp. 19892-19898
- 208. Planes C, Blot-Chabaud M, Matthay MA, et al: Hypoxia and beta 2-agonists regulate cell surface expression of the epithelial sodium channel in native alveolar epithelial cells. J Biol Chem 2002; 277: pp. 47318-47324
- 209. Matthay MA, Brower RG, Carson S, et al: Randomized, placebo-controlled clinical trial of an aerosolized beta(2)-agonist for treatment of acute lung injury. Am J Respir Crit Care Med 2011; 184: pp. 561-568
- 210. Tuder RM, Petrache I, Elias JA, et al: Apoptosis and emphysema: the missing link. Am J Respir Cell Mol Biol 2003; 28: pp. 551-554
- 211. Imai K, Mercer BA, Schulman LL, et al: Correlation of lung surface area to apoptosis and proliferation in human emphysema. Eur Respir J 2005; 25: pp. 250-258
- 212. Aoshiba K, Yokohori N, and Nagai A: Alveolar wall apoptosis causes lung destruction and emphysematous changes. Am J Respir Cell Mol Biol 2003; 28: pp. 555-562
- 213. Hamacher J, Lucas R, Lijnen HR, et al: Tumor necrosis factor-alpha and angiostatin are mediators of endothelial cytotoxicity in bronchoalveolar lavages of patients with acute respiratory distress syndrome. Am J Respir Crit Care Med 2002; 166: pp. 651-656
- 214. Newman V, Gonzalez RF, Matthay MA, et al: A novel alveolar type I cell-specific biochemical marker of human acute lung injury. Am J Respir Crit Care Med 2000; 161: pp. 990-995
- 215. Li X, Shu R, Filippatos G, et al: Apoptosis in lung injury and remodeling. J Appl Physiol 2004; 97: pp. 1535-1542
- 216. Martin TR, Hagimoto N, Nakamura M, et al: Apoptosis and epithelial injury in the lungs. Proc Am Thorac Soc 2005; 2: pp. 214-220
- 217. Adamson IY, and Bowden DH: The type 2 cell as progenitor of alveolar epithelial regeneration. A cytodynamic study in mice after exposure to oxygen. Lab Invest 1974; 30: pp. 35-42
- 218. Berthiaume Y, Lesur O, and Dagenais A: Treatment of adult respiratory distress syndrome: plea for rescue therapy of the alveolar epithelium. Thorax 1999; 54: pp. 150-160
- 219. Galluzzi L, Maiuri MC, Vitale I, et al: Cell death modalities: classification and pathophysiological implications. Cell Death Differ 2007; 14: pp. 1237-1243
- 220. Kim I, Xu W, and Reed JC: Cell death and endoplasmic reticulum stress: disease relevance and therapeutic opportunities. Nat Rev Drug Discov 2008; 7: pp. 1013-1030
- 221. Guinee D, Brambilla E, Fleming M, et al: The potential role of BAX and BCL-2 expression in diffuse alveolar damage. Am J Pathol 1997; 151: pp. 999-1007
- 222. Guinee D, Fleming M, Hayashi T, et al: Association of p53 and WAF1 expression with apoptosis in diffuse alveolar damage. Am J Pathol 1996; 149: pp. 531-538
- 223. Albertine KH, Soulier MF, Wang Z, et al: Fas and fas ligand are up-regulated in pulmonary edema fluid and lung tissue of patients with acute lung injury and the acute respiratory distress syndrome. Am J Pathol 2002; 161: pp. 1783-1796
- 224. Matute-Bello G, Liles WC, Steinberg KP, et al: Soluble Fas ligand induces epithelial cell apoptosis in humans with acute lung injury (ARDS). J Immunol 1999; 163: pp. 2217-2225
- 225. Herrero R, Kajikawa O, Matute-Bello G, et al: The biological activity of FasL in human and mouse lungs is determined by the structure of its stalk region. J Clin Invest 2011; 121: pp. 1174-1190
- 226. Matute-Bello G, Liles WC, Frevert CW, et al: Recombinant human Fas ligand induces alveolar epithelial cell apoptosis and lung injury in rabbits. Am J Physiol Lung Cell Mol Physiol 2001; 281: pp. L328-L335
- 227. Kawasaki M, Kuwano K, Hagimoto N, et al: Protection from lethal apoptosis in lipopolysaccharide-induced acute lung injury in mice by a caspase inhibitor. Am J Pathol 2000; 157: pp. 597-603
- 228. Juncadella IJ, Kadl A, Sharma AK, et al: Apoptotic cell clearance by bronchial epithelial cells critically influences airway inflammation. Nature 2013; 493: pp. 547-551
- 229. Elliott MR, and Ravichandran KS: Clearance of apoptotic cells: implications in health and disease. J Cell Biol 2010; 189: pp. 1059-1070
- 230. Bhandari V, Choo-Wing R, Lee CG, et al: Hyperoxia causes angiopoietin 2-mediated acute lung injury and necrotic cell death. Nat Med 2006; 12: pp. 1286-1293
- 231. Jamieson AM, Pasman L, Yu S, et al: Role of tissue protection in lethal respiratory viral-bacterial coinfection. Science 2013; 340: pp. 1230-1234
- 232. Sznajder JI: Strategies to increase alveolar epithelial fluid removal in the injured lung. Am J Respir Crit Care Med 1999; 160: pp. 1441-1442
- 233. Folkesson HG, Kuzenko SR, Lipson DA, et al: The adenosine 2A receptor agonist GW328267C improves lung function after acute lung injury in rats. Am J Physiol Lung Cell Mol Physiol 2012; 303: pp. L259-L271
- 234. Eckle T, Grenz A, Laucher S, et al: A2B adenosine receptor signaling attenuates acute lung injury by enhancing alveolar fluid clearance in mice. J Clin Invest 2008; 118: pp. 3301-3315
- 235. Johnson MD, Widdicombe JH, Allen L, et al: Alveolar epithelial type I cells contain transport proteins and transport sodium, supporting an active role for type I cells in regulation of lung liquid homeostasis. Proc Natl Acad Sci U S A 2002; 99: pp. 1966-1971
- 236. Borok Z, Hami A, Danto SI, et al: Effects of EGF on alveolar epithelial junctional permeability and active sodium transport. Am J Physiol 1996; 270: pp. L559-L565
- 237. Borok Z, Liebler JM, Lubman RL, et al: Na transport proteins are expressed by rat alveolar epithelial type I cells. Am J Physiol Lung Cell Mol Physiol 2002; 282: pp. L599-L608
- 238. Serhan CN, and Savill J: Resolution of inflammation: the beginning programs the end. Nat Immunol 2005; 6: pp. 1191-1197
- 239. Herridge MS, Tansey CM, Matte A, et al: Functional disability 5 years after acute respiratory distress syndrome. N Engl J Med 2011; 364: pp. 1293-1304
- 240. Garcia JG, Liu F, Verin AD, et al: Sphingosine 1-phosphate promotes endothelial cell barrier integrity by Edg-dependent cytoskeletal rearrangement. J Clin Invest 2001; 108: pp. 689-701
- 241. Ephstein Y, Singleton PA, Chen W, et al: Critical role of S1PR1 and integrin beta4 in HGF/c-Met-mediated increases in vascular integrity. J Biol Chem 2013; 288: pp. 2191-2200
- 242. Zhao Y, Gorshkova IA, Berdyshev E, et al: Protection of LPS-induced murine acute lung injury by sphingosine-1-phosphate lyase suppression. Am J Respir Cell Mol Biol 2011; 45: pp. 426-435
- 243. Su G, Atakilit A, Li JT, et al: Absence of integrin alphavbeta3 enhances vascular leak in mice by inhibiting endothelial cortical actin formation. Am J Respir Crit Care Med 2012; 185: pp. 58-66
- 244. Jones CA, Nishiya N, London NR, et al: Slit2-Robo4 signalling promotes vascular stability by blocking Arf6 activity. Nat Cell Biol 2009; 11: pp. 1325-1331
- 245. London NR, Zhu W, Bozza FA, et al: Targeting Robo4-dependent Slit signaling to survive the cytokine storm in sepsis and influenza. Sci Transl Med 2010; 2: pp. 23ra19
- 246. Kouklis P, Konstantoulaki M, Vogel S, et al: Cdc42 regulates the restoration of endothelial barrier function. Circ Res 2004; 94: pp. 159-166
- 247. Wiener-Kronish JP, Albertine KH, and Matthay MA: Differential responses of the endothelial and epithelial barriers of the lung in sheep to Escherichia coli endotoxin. J Clin Invest 1991; 88: pp. 864-875
- 248. Matthay MA, and Wiener-Kronish JP: Intact epithelial barrier function is critical for the resolution of alveolar edema in humans. Am Rev Respir Dis 1990; 142: pp. 1250-1257
- 249. Ahdieh M, Vandenbos T, and Youakim A: Lung epithelial barrier function and wound healing are decreased by IL-4 and IL-13 and enhanced by IFN-gamma. Am J Physiol Cell Physiol 2001; 281: pp. C2029-C2038
- 250. Miyoshi K, Yanagi S, Kawahara K, et al: Epithelial Pten controls acute lung injury and fibrosis by regulating alveolar epithelial cell integrity. Am J Respir Crit Care Med 2013; 187: pp. 262-275
- 251. Zhao Y, Zhao J, Mialki RK, et al: Lipopolysaccharide-induced phosphorylation of c-Met tyrosine residue 1003 regulates c-Met intracellular trafficking and lung epithelial barrier function. Am J Physiol Lung Cell Mol Physiol 2013; 305: pp. L56-L63
- 252. LaFemina MJ, Rokkam D, Chandrasena A, et al: Keratinocyte growth factor enhances barrier function without altering claudin expression in primary alveolar epithelial cells. Am J Physiol Lung Cell Mol Physiol 2010; 299: pp. L724-L734
- 253. Wray C, Mao Y, Pan J, et al: Claudin-4 augments alveolar epithelial barrier function and is induced in acute lung injury. Am J Physiol Lung Cell Mol Physiol 2009; 297: pp. L219-L227
- 254. Mason RJ, and Williams MC: Type II alveolar cell. Defender of the alveolus. Am Rev Respir Dis 1977; 115: pp. 81-91
- 255. Adamson IY, Hedgecock C, and Bowden DH: Epithelial cell-fibroblast interactions in lung injury and repair. Am J Pathol 1990; 137: pp. 385-392
- 256. Adamson IY, Young L, and Bowden DH: Relationship of alveolar epithelial injury and repair to the induction of pulmonary fibrosis. Am J Pathol 1988; 130: pp. 377-383
- 257. Garat C, Kheradmand F, Albertine KH, et al: Soluble and insoluble fibronectin increases alveolar epithelial wound healing in vitro. Am J Physiol 1996; 271: pp. L844-L853
- 258. Kheradmand F, Folkesson HG, Shum L, et al: Transforming growth factor-alpha enhances alveolar epithelial cell repair in a new in vitro model. Am J Physiol 1994; 267: pp. L728-L738
- 259. Atabai K, Ishigaki M, Geiser T, et al: Keratinocyte growth factor can enhance alveolar epithelial repair by nonmitogenic mechanisms. Am J Physiol Lung Cell Mol Physiol 2002; 283: pp. L163-L169
- 260. Gurtner GC, Werner S, Barrandon Y, et al: Wound repair and regeneration. Nature 2008; 453: pp. 314-321
- 261. Raftopoulou M, and Hall A: Cell migration: Rho GTPases lead the way. Dev Biol 2004; 265: pp. 23-32
- 262. Desai LP, Aryal AM, Ceacareanu B, et al: RhoA and Rac1 are both required for efficient wound closure of airway epithelial cells. Am J Physiol Lung Cell Mol Physiol 2004; 287: pp. L1134-L1144
- 263. Rogel MR, Soni PN, Troken JR, et al: Vimentin is sufficient and required for wound repair and remodeling in alveolar epithelial cells. FASEB J 2011; 25: pp. 3873-3883
- 264. Geiser T, Atabai K, Jarreau PH, et al: Pulmonary edema fluid from patients with acute lung injury augments in vitro alveolar epithelial repair by an IL-1beta-dependent mechanism. Am J Respir Crit Care Med 2001; 163: pp. 1384-1388
- 265. Geiser T, Jarreau PH, Atabai K, et al: Interleukin-1beta augments in vitro alveolar epithelial repair. Am J Physiol Lung Cell Mol Physiol 2000; 279: pp. L1184-L1190
- 266. Buckley S, Shi W, Carraro G, et al: The milieu of damaged alveolar epithelial type 2 cells stimulates alveolar wound repair by endogenous and exogenous progenitors. Am J Respir Cell Mol Biol 2011; 45: pp. 1212-1221
- 267. Desai LP, Chapman KE, and Waters CM: Mechanical stretch decreases migration of alveolar epithelial cells through mechanisms involving Rac1 and Tiam1. Am J Physiol Lung Cell Mol Physiol 2008; 295: pp. L958-L965
- 268. Mihai C, Bao S, Lai JP, et al: PTEN inhibition improves wound healing in lung epithelia through changes in cellular mechanics that enhance migration. Am J Physiol Lung Cell Mol Physiol 2012; 302: pp. L287-L299
- 269. Flozak AS, Lam AP, Russell S, et al: Beta-catenin/T-cell factor signaling is activated during lung injury and promotes the survival and migration of alveolar epithelial cells. J Biol Chem 2010; 285: pp. 3157-3167
- 270. Zemans RL, McClendon J, Aschner Y, et al: Role of beta-catenin-regulated CCN matricellular proteins in epithelial repair after inflammatory lung injury. Am J Physiol Lung Cell Mol Physiol 2013; 304: pp. L415-L427
- 271. Zhu M, Tian D, Li J, et al: Glycogen synthase kinase 3beta and beta-catenin are involved in the injury and repair of bronchial epithelial cells induced by scratching. Exp Mol Pathol 2007; 83: pp. 30-38
- 272. Tanjore H, Degryse AL, Crossno PF, et al: β-Catenin in the alveolar epithelium protects from lung fibrosis following intratracheal bleomycin. Am J Respir Crit Care Med 2013; 187: pp. 630-639
- 273. Altemeier WA, Schlesinger SY, Buell CA, et al: Transmembrane and extracellular domains of syndecan-1 have distinct functions in regulating lung epithelial migration and adhesion. J Biol Chem 2012; 287: pp. 34927-34935
- 274. Chen P, Abacherli LE, Nadler ST, et al: MMP7 shedding of syndecan-1 facilitates re-epithelialization by affecting alpha(2)beta(1) integrin activation. PLoS ONE 2009; 4: pp. e6565
- 275. Gorissen SH, Hristova M, Habibovic A, et al: Dual oxidase-1 is required for airway epithelial cell migration and bronchiolar reepithelialization after injury. Am J Respir Cell Mol Biol 2013; 48: pp. 337-345
- 276. Wesley UV, Bove PF, Hristova M, et al: Airway epithelial cell migration and wound repair by ATP-mediated activation of dual oxidase 1. J Biol Chem 2007; 282: pp. 3213-3220
- 277. Crosby LM, and Waters CM: Epithelial repair mechanisms in the lung. Am J Physiol Lung Cell Mol Physiol 2010; 298: pp. L715-L731
- 278. Horan GS, Wood S, Ona V, et al: Partial inhibition of integrin alpha(v)beta6 prevents pulmonary fibrosis without exacerbating inflammation. Am J Respir Crit Care Med 2008; 177: pp. 56-65
- 279. Hynes RO: Integrins: bidirectional, allosteric signaling machines. Cell 2002; 110: pp. 673-687
- 280. Kim HJ, Henke CA, Savik SK, et al: Integrin mediation of alveolar epithelial cell migration on fibronectin and type I collagen. Am J Physiol 1997; 273: pp. L134-L141
- 281. Kim HJ, Ingbar DH, and Henke CA: Integrin mediation of type II cell adherence to provisional matrix proteins. Am J Physiol 1996; 271: pp. L277-L286
- 282. Aoshiba K, Rennard SI, and Spurzem JR: Fibronectin supports bronchial epithelial cell adhesion and survival in the absence of growth factors. Am J Physiol 1997; 273: pp. L684-L693
- 283. Crosby LM, Luellen C, Zhang Z, et al: Balance of life and death in alveolar epithelial type II cells: proliferation, apoptosis, and the effects of cyclic stretch on wound healing. Am J Physiol Lung Cell Mol Physiol 2011; 301: pp. L536-L546
- 284. Kasper M, and Haroske G: Alterations in the alveolar epithelium after injury leading to pulmonary fibrosis. Histol Histopathol 1996; 11: pp. 463-483
- 285. Rock JR, Barkauskas CE, Cronce MJ, et al: Multiple stromal populations contribute to pulmonary fibrosis without evidence for epithelial to mesenchymal transition. Proc Natl Acad Sci U S A 2011; 108: pp. E1475-E1483
- 286. Panos RJ, Rubin JS, Csaky KG, et al: Keratinocyte growth factor and hepatocyte growth factor/scatter factor are heparin-binding growth factors for alveolar type II cells in fibroblast-conditioned medium. J Clin Invest 1993; 92: pp. 969-977
- 287. Mason RJ, Leslie CC, McCormick-Shannon K, et al: Hepatocyte growth factor is a growth factor for rat alveolar type II cells. Am J Respir Cell Mol Biol 1994; 11: pp. 561-567
- 288. Leslie CC, McCormick-Shannon K, Shannon JM, et al: Heparin-binding EGF-like growth factor is a mitogen for rat alveolar type II cells. Am J Respir Cell Mol Biol 1997; 16: pp. 379-387
- 289. Yano T, Mason RJ, Pan T, et al: KGF regulates pulmonary epithelial proliferation and surfactant protein gene expression in adult rat lung. Am J Physiol Lung Cell Mol Physiol 2000; 279: pp. L1146-L1158
- 290. Morikawa O, Walker TA, Nielsen LD, et al: Effect of adenovector-mediated gene transfer of keratinocyte growth factor on the proliferation of alveolar type II cells in vitro and in vivo. Am J Respir Cell Mol Biol 2000; 23: pp. 626-635
- 291. Mason RJ, McCormick-Shannon K, Rubin JS, et al: Hepatocyte growth factor is a mitogen for alveolar type II cells in rat lavage fluid. Am J Physiol 1996; 271: pp. L46-L53
- 292. Shiratori M, Michalopoulos G, Shinozuka H, et al: Hepatocyte growth factor stimulates DNA synthesis in alveolar epithelial type II cells in vitro. Am J Respir Cell Mol Biol 1995; 12: pp. 171-180
- 293. Panos RJ, Bak PM, Simonet WS, et al: Intratracheal instillation of keratinocyte growth factor decreases hyperoxia-induced mortality in rats. J Clin Invest 1995; 96: pp. 2026-2033
- 294. Ray P, Devaux Y, Stolz DB, et al: Inducible expression of keratinocyte growth factor (KGF) in mice inhibits lung epithelial cell death induced by hyperoxia. Proc Natl Acad Sci U S A 2003; 100: pp. 6098-6103
- 295. Huffman Reed JA, Rice WR, Zsengeller ZK, et al: GM-CSF enhances lung growth and causes alveolar type II epithelial cell hyperplasia in transgenic mice. Am J Physiol 1997; 273: pp. L715-L725
- 296. Cakarova L, Marsh LM, Wilhelm J, et al: Macrophage tumor necrosis factor-alpha induces epithelial expression of granulocyte-macrophage colony-stimulating factor: impact on alveolar epithelial repair. Am J Respir Crit Care Med 2009; 180: pp. 521-532
- 297. Standiford LR, Standiford TJ, Newstead MJ, et al: TLR4-dependent GM-CSF protects against lung injury in gram-negative bacterial pneumonia. Am J Physiol Lung Cell Mol Physiol 2012; 302: pp. L447-L454
- 298. Huang FF, Barnes PF, Feng Y, et al: GM-CSF in the lung protects against lethal influenza infection. Am J Respir Crit Care Med 2011; 184: pp. 259-268
- 299. Sturrock A, Seedahmed E, Mir-Kasimov M, et al: GM-CSF provides autocrine protection for murine alveolar epithelial cells from oxidant-induced mitochondrial injury. Am J Physiol Lung Cell Mol Physiol 2012; 302: pp. L343-L351
- 300. Konigshoff M, Kramer M, Balsara N, et al: WNT1-inducible signaling protein-1 mediates pulmonary fibrosis in mice and is upregulated in humans with idiopathic pulmonary fibrosis. J Clin Invest 2009; 119: pp. 772-787
- 301. Zemans RL, Briones N, Campbell M, et al: Neutrophil transmigration triggers repair of the lung epithelium via beta-catenin signaling. Proc Natl Acad Sci U S A 2011; 108: pp. 15990-15995
- 302. Marsh LM, Cakarova L, Kwapiszewska G, et al: Surface expression of CD74 by type II alveolar epithelial cells: a potential mechanism for macrophage migration inhibitory factor-induced epithelial repair. Am J Physiol Lung Cell Mol Physiol 2009; 296: pp. L442-L452
- 303. Liu Y, Sadikot RT, Adami GR, et al: FoxM1 mediates the progenitor function of type II epithelial cells in repairing alveolar injury induced by Pseudomonas aeruginosa. J Exp Med 2011; 208: pp. 1473-1484
- 304. Adamson IY, and Bowden DH: Derivation of type 1 epithelium from type 2 cells in the developing rat lung. Lab Invest 1975; 32: pp. 736-745
- 305. Barkauskas CE, Cronce MJ, Rackley CR, et al: Type 2 alveolar cells are stem cells in adult lung. J Clin Invest 2013; 123: pp. 3025-3036
- 305a. Desai TJ, Brownfield DG, and Krasnow MA: Alveolar progenitor and stem cells in lung development, renewal and cancer. Nature 2014; 507: pp. 190-194
- 306. Bardales RH, Xie SS, Schaefer RF, et al: Apoptosis is a major pathway responsible for the resolution of type II pneumocytes in acute lung injury. Am J Pathol 1996; 149: pp. 845-852
- 307. Fehrenbach H, Kasper M, Tschernig T, et al: Keratinocyte growth factor-induced hyperplasia of rat alveolar type II cells in vivo is resolved by differentiation into type I cells and by apoptosis. Eur Respir J 1999; 14: pp. 534-544
- 308. Zhao L, Yee M, and O’Reilly MA: Transdifferentiation of alveolar epithelial type II to type I cells is controlled by opposing TGF-beta and BMP signaling. Am J Physiol Lung Cell Mol Physiol 2013; 305: pp. L409-L418
- 309. Ghosh MC, Gorantla V, Makena PS, et al: Insulin-like growth factor-I stimulates differentiation of ATII cells to ATI-like cells through activation of Wnt5a. Am J Physiol Lung Cell Mol Physiol 2013; 305: pp. L222-L228
- 310. Danto SI, Shannon JM, Borok Z, et al: Reversible transdifferentiation of alveolar epithelial cells. Am J Respir Cell Mol Biol 1995; 12: pp. 497-502
- 311. Gonzalez RF, Allen L, and Dobbs LG: Rat alveolar type I cells proliferate, express OCT-4, and exhibit phenotypic plasticity in vitro. Am J Physiol Lung Cell Mol Physiol 2009; 297: pp. L1045-L1055
- 312. Lawson WE, Cheng DS, Degryse AL, et al: Endoplasmic reticulum stress enhances fibrotic remodeling in the lungs. Proc Natl Acad Sci U S A 2011; 108: pp. 10562-10567
- 313. Sisson TH, Mendez M, Choi K, et al: Targeted injury of type II alveolar epithelial cells induces pulmonary fibrosis. Am J Respir Crit Care Med 2010; 181: pp. 254-263
- 314. Kuwano K, Hagimoto N, Kawasaki M, et al: Essential roles of the Fas-Fas ligand pathway in the development of pulmonary fibrosis. J Clin Invest 1999; 104: pp. 13-19
- 315. Martin C, Papazian L, Payan MJ, et al: Pulmonary fibrosis correlates with outcome in adult respiratory distress syndrome. A study in mechanically ventilated patients. Chest 1995; 107: pp. 196-200
- 316. Marshall RP, Bellingan G, Webb S, et al: Fibroproliferation occurs early in the acute respiratory distress syndrome and impacts on outcome. Am J Respir Crit Care Med 2000; 162: pp. 1783-1788
- 317. Burnham EL, Janssen WJ, Riches DW, et al: The fibroproliferative response in acute respiratory distress syndrome: mechanisms and clinical significance. Eur Respir J 2013; 43: pp. 276-285
- 318. Young LR, Gulleman PM, Bridges JP, et al: The alveolar epithelium determines susceptibility to lung fibrosis in Hermansky-Pudlak syndrome. Am J Respir Crit Care Med 2012; 186: pp. 1014-1024
- 319. Fernandez IE, and Eickelberg O: New cellular and molecular mechanisms of lung injury and fibrosis in idiopathic pulmonary fibrosis. Lancet 2012; 380: pp. 680-688
- 320. Serrano-Mollar A, Nacher M, Gay-Jordi G, et al: Intratracheal transplantation of alveolar type II cells reverses bleomycin-induced lung fibrosis. Am J Respir Crit Care Med 2007; 176: pp. 1261-1268
- 321. Puchelle E, Zahm JM, Tournier JM, et al: Airway epithelial repair, regeneration, and remodeling after injury in chronic obstructive pulmonary disease. Proc Am Thorac Soc 2006; 3: pp. 726-733
- 322. Uhal BD: Epithelial apoptosis in the initiation of lung fibrosis. Eur Respir J Suppl 2003; 44: pp. 7s-9s
- 323. Yano T, Deterding RR, Simonet WS, et al: Keratinocyte growth factor reduces lung damage due to acid instillation in rats. Am J Respir Cell Mol Biol 1996; 15: pp. 433-442
- 324. Garibaldi BT, D’Alessio FR, Mock JR, et al: Regulatory T cells reduce acute lung injury fibroproliferation by decreasing fibrocyte recruitment. Am J Respir Cell Mol Biol 2013; 48: pp. 35-43
- 325. Zemke AC, Teisanu RM, Giangreco A, et al: beta-Catenin is not necessary for maintenance or repair of the bronchiolar epithelium. Am J Respir Cell Mol Biol 2009; 41: pp. 535-543
- 326. Henderson WR, Chi EY, Ye X, et al: Inhibition of Wnt/beta-catenin/CREB binding protein (CBP) signaling reverses pulmonary fibrosis. Proc Natl Acad Sci U S A 2010; 107: pp. 14309-14314
- 327. Zhou B, Liu Y, Kahn M, et al: Interactions between beta-catenin and transforming growth factor-beta signaling pathways mediate epithelial-mesenchymal transition and are dependent on the transcriptional co-activator cAMP-response element-binding protein (CREB)-binding protein (CBP). J Biol Chem 2012; 287: pp. 7026-7038
- 328. Beers MF, and Morrisey EE: The three R’s of lung health and disease: repair, remodeling, and regeneration. J Clin Invest 2011; 121: pp. 2065-2073
- 329. Rossi AG, Sawatzky DA, Walker A, et al: Cyclin-dependent kinase inhibitors enhance the resolution of inflammation by promoting inflammatory cell apoptosis. Nat Med 2006; 12: pp. 1056-1064
- 330. Walmsley SR, Chilvers ER, Thompson AA, et al: Prolyl hydroxylase 3 (PHD3) is essential for hypoxic regulation of neutrophilic inflammation in humans and mice. J Clin Invest 2011; 121: pp. 1053-1063
- 331. Walmsley SR, Print C, Farahi N, et al: Hypoxia-induced neutrophil survival is mediated by HIF-1alpha-dependent NF-kappaB activity. J Exp Med 2005; 201: pp. 105-115
- 332. D’Alessio FR, Tsushima K, Aggarwal NR, et al: CD4+CD25+Foxp3+ Tregs resolve experimental lung injury in mice and are present in humans with acute lung injury. J Clin Invest 2009; 119: pp. 2898-2913
- 333. Hoffmann PR, deCathelineau AM, Ogden CA, et al: Phosphatidylserine (PS) induces PS receptor-mediated macropinocytosis and promotes clearance of apoptotic cells. J Cell Biol 2001; 155: pp. 649-659
- 334. Gardai SJ, McPhillips KA, Frasch SC, et al: Cell-surface calreticulin initiates clearance of viable or apoptotic cells through trans-activation of LRP on the phagocyte. Cell 2005; 123: pp. 321-334
- 335. Ogden CA, deCathelineau A, Hoffmann PR, et al: C1q and mannose binding lectin engagement of cell surface calreticulin and CD91 initiates macropinocytosis and uptake of apoptotic cells. J Exp Med 2001; 194: pp. 781-795
- 336. Teder P, Vandivier RW, Jiang D, et al: Resolution of lung inflammation by CD44. Science 2002; 296: pp. 155-158
- 337. Park D, Han CZ, Elliott MR, et al: Continued clearance of apoptotic cells critically depends on the phagocyte Ucp2 protein. Nature 2011; 477: pp. 220-224
- 338. Banerjee S, de Freitas A, Friggeri A, et al: Intracellular HMGB1 negatively regulates efferocytosis. J Immunol 2011; 187: pp. 4686-4694
- 339. Yang Y, Friggeri A, Banerjee S, et al: Urokinase-type plasminogen activator inhibits efferocytosis of neutrophils. Am J Respir Crit Care Med 2010; 182: pp. 1516-1523
- 340. Fadok VA, Bratton DL, Konowal A, et al: Macrophages that have ingested apoptotic cells in vitro inhibit proinflammatory cytokine production through autocrine/paracrine mechanisms involving TGF-beta, PGE2, and PAF. J Clin Invest 1998; 101: pp. 890-898
- 341. Poe SL, Arora M, Oriss TB, et al: STAT1-regulated lung MDSC-like cells produce IL-10 and efferocytose apoptotic neutrophils with relevance in resolution of bacterial pneumonia. Mucosal Immunol 2013; 6: pp. 189-199
- 342. Matute-Bello G, Liles WC, Radella F, et al: Neutrophil apoptosis in the acute respiratory distress syndrome. Am J Respir Crit Care Med 1997; 156: pp. 1969-1977
- 343. Haslett C: Granulocyte apoptosis and its role in the resolution and control of lung inflammation. Am J Respir Crit Care Med 1999; 160: pp. S5-S11
- 344. Vandivier RW, Henson PM, and Douglas IS: Burying the dead: the impact of failed apoptotic cell removal (efferocytosis) on chronic inflammatory lung disease. Chest 2006; 129: pp. 1673-1682
- 345. El Kebir D, Gjorstrup P, and Filep JG: Resolvin E1 promotes phagocytosis-induced neutrophil apoptosis and accelerates resolution of pulmonary inflammation. Proc Natl Acad Sci U S A 2012; 109: pp. 14983-14988
- 346. Godson C, Mitchell S, Harvey K, et al: Cutting edge: lipoxins rapidly stimulate nonphlogistic phagocytosis of apoptotic neutrophils by monocyte-derived macrophages. J Immunol 2000; 164: pp. 1663-1667
- 347. Morimoto K, Janssen WJ, Fessler MB, et al: Lovastatin enhances clearance of apoptotic cells (efferocytosis) with implications for chronic obstructive pulmonary disease. J Immunol 2006; 176: pp. 7657-7665
- 348. Frasch SC, Fernandez-Boyanapalli RF, Berry KA, et al: Neutrophils regulate tissue neutrophilia in inflammation via the oxidant-modified lipid lysophosphatidylserine. J Biol Chem 2013; 288: pp. 4583-4593
- 349. Uddin M, and Levy BD: Resolvins: natural agonists for resolution of pulmonary inflammation. Prog Lipid Res 2011; 50: pp. 75-88
- 350. Bonnans C, and Levy BD: Lipid mediators as agonists for the resolution of acute lung inflammation and injury. Am J Respir Cell Mol Biol 2007; 36: pp. 201-205
- 351. Planaguma A, Pfeffer MA, Rubin G, et al: Lovastatin decreases acute mucosal inflammation via 15-epi-lipoxin A(4). Mucosal Immunol 2010; 3: pp. 270-279
- 352. Serhan CN, Maddox JF, Petasis NA, et al: Design of lipoxin A4 stable analogs that block transmigration and adhesion of human neutrophils. Biochemistry 1995; 34: pp. 14609-14615
- 353. Janssen WJ, Barthel L, Muldrow A, et al: Fas determines differential fates of resident and recruited macrophages during resolution of acute lung injury. Am J Respir Crit Care Med 2011; 184: pp. 547-560
- 354. Morrison SJ: Stem cell potential: can anything make anything? Curr Biol 2001; 11: pp. R7-R9
- 355. Engelhardt JF: Stem cell niches in the mouse airway. Am J Respir Cell Mol Biol 2001; 24: pp. 649-652
- 356. Stripp BR, and Reynolds SD: Maintenance and repair of the bronchiolar epithelium. Proc Am Thorac Soc 2008; 5: pp. 328-333
- 357. Bertoncello I, and McQualter JL: Lung stem cells: do they exist? Respirology 2013; 18: pp. 587-595
- 358. Kumar PA, Hu Y, Yamamoto Y, et al: Distal airway stem cells yield alveoli in vitro and during lung regeneration following H1N1 influenza infection. Cell 2011; 147: pp. 525-538
- 359. Kim CF, Jackson EL, Woolfenden AE, et al: Identification of bronchioalveolar stem cells in normal lung and lung cancer. Cell 2005; 121: pp. 823-835
- 360. Wansleeben C, Barkauskas CE, Rock JR, et al: Stem cells of the adult lung: their development and role in homeostasis, regeneration, and disease. Wiley Interdiscip Rev Dev Biol 2013; 2: pp. 131-148
- 361. Zheng D, Limmon GV, Yin L, et al: Regeneration of alveolar type I and II cells from Scgb1a1-expressing cells following severe pulmonary damage induced by bleomycin and influenza. PLoS ONE 2012; 7: pp. e48451
- 362. Chapman HA, Li X, Alexander JP, et al: Integrin alpha6beta4 identifies an adult distal lung epithelial population with regenerative potential in mice. J Clin Invest 2011; 121: pp. 2855-2862
- 363. Evans MJ, Cabral-Anderson LJ, and Freeman G: Role of the Clara cell in renewal of the bronchiolar epithelium. Lab Invest 1978; 38: pp. 648-653
- 364. Evans MJ, Dekker NP, Cabral-Anderson LJ, et al: Quantitation of damage to the alveolar epithelium by means of type 2 cell proliferation. Am Rev Respir Dis 1978; 118: pp. 787-790
- 365. Evans MJ, Johnson LV, Stephens RJ, et al: Renewal of the terminal bronchiolar epithelium in the rat following exposure to NO2 or O3. Lab Invest 1976; 35: pp. 246-257
- 366. Evans MJ, Shami SG, Cabral-Anderson LJ, et al: Role of nonciliated cells in renewal of the bronchial epithelium of rats exposed to NO2. Am J Pathol 1986; 123: pp. 126-133
- 367. Plopper CG, Macklin J, Nishio SJ, et al: Relationship of cytochrome P-450 activity to Clara cell cytotoxicity. III. Morphometric comparison of changes in the epithelial populations of terminal bronchioles and lobar bronchi in mice, hamsters, and rats after parenteral administration of naphthalene. Lab Invest 1992; 67: pp. 553-565
- 368. Plopper CG, Suverkropp C, Morin D, et al: Relationship of cytochrome P-450 activity to Clara cell cytotoxicity. I. Histopathologic comparison of the respiratory tract of mice, rats and hamsters after parenteral administration of naphthalene. J Pharmacol Exp Ther 1992; 261: pp. 353-363
- 369. Buckpitt A, Buonarati M, Avey LB, et al: Relationship of cytochrome P450 activity to Clara cell cytotoxicity. II. Comparison of stereoselectivity of naphthalene epoxidation in lung and nasal mucosa of mouse, hamster, rat and rhesus monkey. J Pharmacol Exp Ther 1992; 261: pp. 364-372
- 370. Larson SD, Plopper CG, Baker G, et al: Proximal airway mucous cells of ovalbumin-sensitized and -challenged Brown Norway rats accumulate the neuropeptide calcitonin gene-related peptide. Am J Physiol Lung Cell Mol Physiol 2004; 287: pp. L286-L295
- 371. Evans CM, Williams OW, Tuvim MJ, et al: Mucin is produced by Clara cells in the proximal airways of antigen-challenged mice. Am J Respir Cell Mol Biol 2004; 31: pp. 382-394
- 372. Tesfaigzi Y: Roles of apoptosis in airway epithelia. Am J Respir Cell Mol Biol 2006; 34: pp. 537-547
- 373. Jeffery PK: Remodeling in asthma and chronic obstructive lung disease. Am J Respir Crit Care Med 2001; 164: pp. S28-S38
- 374. Shijubo N, Itoh Y, Yamaguchi T, et al: Clara cell protein-positive epithelial cells are reduced in small airways of asthmatics. Am J Respir Crit Care Med 1999; 160: pp. 930-933
- 375. Mattsson J, Remberger M, Andersson O, et al: Decreased serum levels of Clara cell secretory protein (CC16) are associated with bronchiolitis obliterans and may permit early diagnosis in patients after allogeneic stem-cell transplantation. Transplantation 2005; 79: pp. 1411-1416
- 376. Zhang Y, Wroblewski M, Hertz MI, et al: Analysis of chronic lung transplant rejection by MALDI-TOF profiles of bronchoalveolar lavage fluid. Proteomics 2006; 6: pp. 1001-1010
- 377. Engelhardt JF, Schlossberg H, Yankaskas JR, et al: Progenitor cells of the adult human airway involved in submucosal gland development. Development 1995; 121: pp. 2031-2046
- 378. Miller YE, Blatchford P, Hyun DS, et al: Bronchial epithelial Ki-67 index is related to histology, smoking, and gender, but not lung cancer or chronic obstructive pulmonary disease. Cancer Epidemiol Biomarkers Prev 2007; 16: pp. 2425-2431
- 379. Broeckaert F, Clippe A, Knoops B, et al: Clara cell secretory protein (CC16): features as a peripheral lung biomarker. Ann N Y Acad Sci 2000; 923: pp. 68-77
- 380. Mutti A, Corradi M, Goldoni M, et al: Exhaled metallic elements and serum pneumoproteins in asymptomatic smokers and patients with COPD or asthma. Chest 2006; 129: pp. 1288-1297
- 381. Szefler SJ: Asthma progression: can we and should we measure it? J Allergy Clin Immunol 2008; 121: pp. 598-600
- 382. Bisgaard H, and Szefler S: Prevalence of asthma-like symptoms in young children. Pediatr Pulmonol 2007; 42: pp. 723-728
- 383. Rabinovitch N, Strand M, and Gelfand EW: Particulate levels are associated with early asthma worsening in children with persistent disease. Am J Respir Crit Care Med 2006; 173: pp. 1098-1105
- 384. Chilosi M, Poletti V, Zamo A, et al: Aberrant Wnt/beta-catenin pathway activation in idiopathic pulmonary fibrosis. Am J Pathol 2003; 162: pp. 1495-1502
- 385. Voynow JA, Fischer BM, Roberts BC, et al: Basal-like cells constitute the proliferating cell population in cystic fibrosis airways. Am J Respir Crit Care Med 2005; 172: pp. 1013-1018
- 386. Wang H, Liu X, Umino T, et al: Cigarette smoke inhibits human bronchial epithelial cell repair processes. Am J Respir Cell Mol Biol 2001; 25: pp. 772-779
- 387. Cardoso WV: Molecular regulation of lung development. Annu Rev Physiol 2001; 63: pp. 471-494
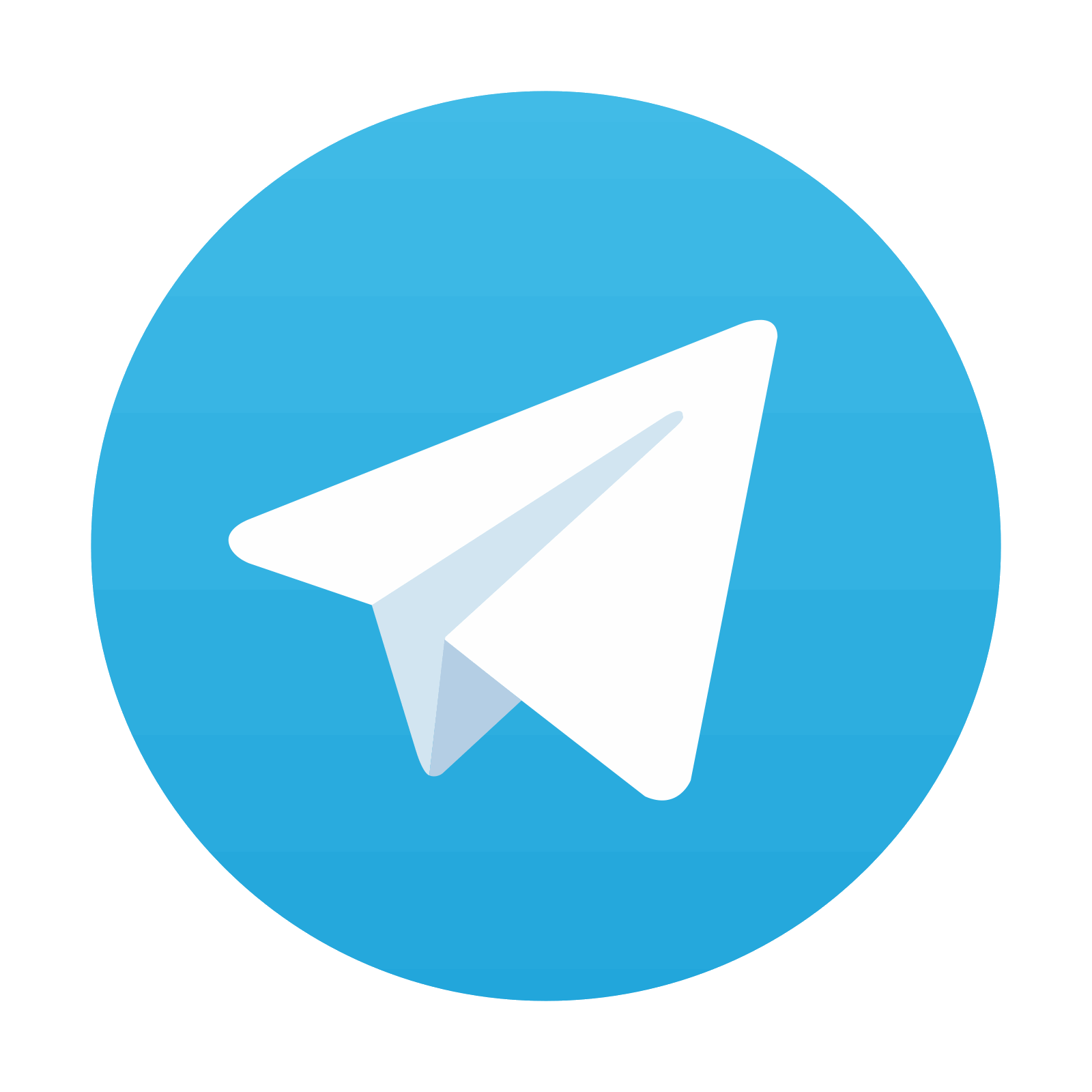
Stay updated, free articles. Join our Telegram channel
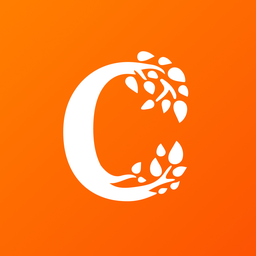
Full access? Get Clinical Tree
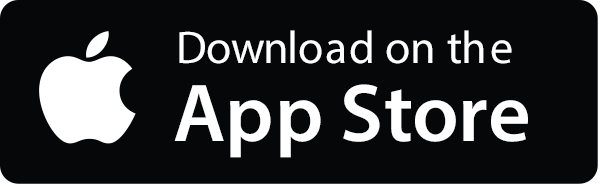
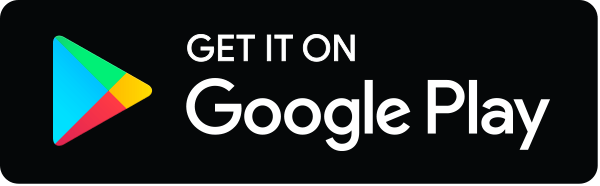