Chapter 84 Implantable Cardioverter-Defibrillators
Device Technology and Implantation Techniques
Introduction
The implantable cardioverter-defibrillator (ICD) has emerged as the primary therapeutic option for the treatment of patients at risk for sudden cardiac death (SCD) caused by ventricular tachyarrhythmias.1–4 ICD devices were originally developed for the secondary prevention of SCD in patients with malignant ventricular arrhythmias and in survivors of SCD. Increasingly, ICDs are used for the primary prevention of SCD in an ever-expanding list of at-risk patient subgroups, which are discussed in this chapter. In addition, ICD systems have undergone a rapid technologic evolution to improve functionality and decrease the morbidity and mortality associated with implantation. These refinements in ICD lead and generator technology have led to a progressive expansion in their use. However, optimal device performance and follow-up require an intimate knowledge of these devices and their operations as well as the clinical electrophysiology of the arrhythmia being managed. This chapter summarizes current ICD technology and insertion techniques for ICD systems when used for SCD prevention.
Implantable Cardioverter-Defibrillator Pulse Generators
The essential elements and principles of these components are detailed in this section. A schematic diagram showing these components is shown in Figure 84-1, A.
Batteries
ICD batteries have several requirements. These include, among others, availability of different voltage levels for pacing and monitoring functions versus defibrillation therapy, substantial longevity, mechanical and electrical durability, and patient safety. In typical ICD batteries, a lithium metal plate serves as the anode, and a silver vanadium oxide plate serves as the cathode (see Figure 84-1). The electrolyte is a solution of a lithium compound in a highly conductive organic solvent or solvents. Multiple layers of the anode and cathode are present in an ICD battery. The battery’s electrode and electrolyte are contained within a hermetically sealed metal case. These two elements immediately interact, and the electrons released from the lithium anode enter the external device circuit. The cathode accepts electrons from the external circuit. Progressive and uncontrolled charge buildup on the anode and cathode is prevented by the ionic nature of the electrolyte.
High-Voltage Shock Therapy
High-voltage capacitors store and deliver the high-energy pulses needed to cardiovert and defibrillate tachyarrhythmias (see Figure 84-1). Capacitors, in essence, consist of two conductors, insulated from each other by a di-electric material, that charge via a charging circuit connected to the battery. During charging, these conductors assume equal but opposite charges that discharge through the lead system to deliver a high-voltage, high-energy shock. Voltage delivered in the shock decays in an exponential waveform, and the actual delivered energy is usually less than the stored energy. Thus measures of both are available for ICD devices. Capacitor size is a major determinant of overall ICD generator size. High-energy density minimizes capacitor size. ICD capacitors use aluminum or tantalum electrode either as foils, which are etched to increase surface area, or as porous pellets. Tantalum capacitors have a smaller size but a greater mass.
Sense Amplifiers and Sensing Circuits
Accurate sensing of input signals and their analysis are important challenges for ICD devices. Near-field and far-field signals are often present in the signal presented by the lead or leads connected to the generator. The amplitude of the signal is measured against a comparator set by the sensing threshold value. However, the tachycardia and fibrillation signal can be highly variable with respect to amplitude on a beat-to-beat basis, along with changing slew rates and morphology. To address this challenge, an important technical feature of ICD sensing circuits is the automatic adjusting signal amplifier “autogain” feature. This feature compensates for variable amplitudes of electrograms during the arrhythmia. The signal amplitude can vary as much as 10 times during a single episode of ventricular fibrillation (VF). Variability in electrocardiogram (ECG) amplitude during VF has also been observed to be a cause for redetection failure.5 The sense amplifier must be able to respond to the widely varying cardiac signals and does so by changing the sensing threshold on a beat-to-beat basis. It is initially set to a fraction of the signal amplitude, and then the threshold decays over time, depending on programming on the threshold and delay. Raising gain for low-amplitude signals can increase oversensing, resulting in oversensing of T waves and “double counting” of fragmented potentials or skeletal myopotentials, leading to inaccurate detection. The autogain feature adjusts sensitivity on the basis of the amplitude of recently sensed signals. The autogain feature of some devices increases sensitivity when a rapid rhythm is detected to sense VF. It also increases sensitivity during bradycardia pacing so that low-amplitude signals from VF will not be interpreted as bradycardia.
Developments in Device Material Technology
Implantable Cardioverter-Defibrillators for Ventricular Defibrillation
Technological developments in the original ICD device have now improved its functionality. The transition from an epicardial lead system to wholly nonthoracotomy placement occurred in 1987, with the inclusion of a left extra-thoracic patch lead that permitted a larger electrode surface area combined with a new left-to-right shock current vector for successful endocardial defibrillation.6 In the original version, a three-electrode configuration permitted two simultaneous shock vectors, left-to-right and right-to-left, for bi-directional current delivery to improve defibrillation thresholds (DFTs). Refinements to both generator and lead systems in the past decade have simplified the implementation of endocardial defibrillation with wholly transvenous implantation. This included use of a biphasic shock waveform as the standard defibrillation shock waveform, which helped reduce defibrillation energy and reduced the need for multiple electrodes for optimal energy transfer to the myocardium.7 New lead configurations have permitted reliable transvenous defibrillation within the maximal leading edge shock voltage (750 to 800 V), available in most ICD generators, in more than 98% of all patients.8 The device can now serve as an integral component of the shock energy delivery system—becoming an active pectoral lead replacing left thoracic patch electrode insertion in the original systems.7 ICD generator size has consistently been reduced, with device volumes now well under 40 cm3 (see Figure 84-1). Although sub-30-cm3 devices are a reality (e.g., the 29-cm3 Sorin Ovatio CRT ICD; Sorin Group, Milan, Italy), the trade-off between generator size and the surface area needed for optimal DFTs is now becoming an issue (see Figure 84-1). Wireless telemetry is now incorporated in three devices currently available in the United States (Biotronik Lumax [Berlin] Medtronic Secura series [Minneapolis, MN], and St Jude Current Promote RF [St Paul, MN]). Because of this feature, the header size increases with overall increase in generator volume (see Figure 84-1).
Single-Chamber Ventricular Implantable Cardioverter-Defibrillator Technology
Device Insertion
In its simplest iteration, the ICD device has capabilities for the detection of lethal ventricular tachyarrhythmias and permits ventricular pacing and monitoring of ventricular rhythms alone. This requires insertion of a single, combined ventricular pacing and defibrillation lead via cephalic or subclavian vein placement with a pectoral generator site—the generator being an active defibrillation shock electrode (Figure 84-2, A). The defibrillation lead includes a large surface area electrode in the right ventricle, integrated or true bipolar sensing, and an active or passive fixation mechanism.9 The shock current is transmitted between the right ventricular electrode and the generator, since a right-to-left shock vector reduces defibrillation threshold energy. Left-sided placement of the generator is therefore preferred, whenever feasible, although right-sided placement is possible. In studies on human DFTs with different lead configurations, the mean energy for defibrillation varies with electrode configuration and location. In addition, the electrode surface area is a critical determinant of current and voltage requirements for defibrillation by virtue of its effects on the electrode-myocardium interface and shock energy vector. Thus DFTs vary in inverse proportion to the electrode surface area, with defined boundaries for such effects in either direction. The addition of a third SVC or right atrial electrode permitted bi-directional shock vectors, which may reduce mean DFTs by 10% to 20%, as shown in some studies, and by larger increments in individual patients. Initially, a second superior venocaval electrode was used for this purpose (see Figure 84-2, B), but now dual-electrode catheters with variable spacing of the two defibrillation coils obviate the need for an additional lead. Similarly, reversal of shock polarity may not reduce DFTs in population studies but can alter thresholds in specific patients. Both these maneuvers are of value in patients with high DFTs. Several endocardial defibrillation leads routinely include two defibrillation electrodes for right ventricular and SVC locations, resulting in a bi-directional shock configuration. Reduction in generator size for cosmetic acceptability and implant simplicity may require attention to optimal generator location for successful and low DFTs. In our early studies on left-sided electrode location for defibrillation, the lowest DFTs were achieved with left axillary locations in the anterior to midaxillary line in the vicinity of the fourth intercostal space, with slightly higher values in the left infraclavicular location in the second intercostal space.10 Currently, this latter location is most commonly used for generator placement. Axillary locations can reduce DFTs, but are not widely used because of arm movement issues; however, they remain an option in some patients. Highest thresholds are obtained with an apical placement in the left midclavicular line at the fourth or fifth intercostal space. Thus the thoracic site of placement or relocation of a generator can have an impact on DFTs, which should be considered by an implanting physician.
Tachyarrhythmia Detection
Ventricular rhythm detection in these systems is based on the ventricular electrogram rate, regularity, morphology, and patterns of electrogram interval changes. Initial detection in all devices is based on absolute ventricular rate, with most devices allowing up to three zones for distinguishing different tachyarrhythmias. In general, a minimum of two-zone programming is usually performed for distinguishing monomorphic ventricular tachycardia (VT) from VF. When empiric programming is sought, we prefer to establish three zones for “slow” VT, “fast” monomorphic ventricular tachyarrhythmias, and VF, as illustrated in Figure 84-3.11–12 Such distinction is useful for both clinical and therapeutic purposes. The slowest zone is usually associated with nonsyncopal rhythms, which are often responsive to anti-tachycardia pacing. The second zone has symptomatic rhythms, but early anti-tachycardia pacing or cardioversion with a lower energy shock with rapid charge times may abort the syncope. Very rapid anti-tachycardia pacing can be considered in this zone. Finally, ventricular fibrillation is usually syncopal in many patients and requires a highly effective shock for immediate termination based on threshold determination. Although nominal detection rate values are provided by manufacturers, it is important to individualize these values on the basis of the patient’s sinus rhythm mechanism, its chronotropic competence, exercise response and activity level of the patient, and the presence of coexisting supraventricular tachyarrhythmias and their ventricular rates. It is generally preferable to ensure a difference of 15 to 20 beats/min between the maximal sinus rate or supraventricular arrhythmia rate and the initial threshold rate for VT detection, provided the latter arrhythmia rate falls above this value by at least 10 to 15 beats/min. If this level of distinction is not possible on the basis of rate alone, and overlapping rates are present between supraventricular tachycardias (SVTs) and VTs, other algorithms are available in most devices to help identify the arrhythmia.
All devices offer sudden onset criteria, which identify an abrupt cycle length change in the ventricular cycle, to discriminate a pathologic tachycardia from sinus tachycardia. In addition, electrogram morphology in supraventricular and ventricular tachyarrhythmias may be matched to the sinus rhythm template. In the absence of intraventricular conduction abnormalities, supraventricular rhythms can often be identified by similar ventricular electrogram morphology. The duration of the ventricular electrogram as a discriminating factor, although used often, is less valuable. Ventricular electrogram duration may increase in ventricular tachyarrhythmias because of slower intraventricular conduction without the use of the specialized conduction system (Figure 84-4). However, few systematic data validate this belief, and “narrow” complex VTs are surprisingly common. Most devices consider sustained high-rate events as ventricular in origin for patient safety and trigger a therapeutic strategy. In many patients, ventricular tachyarrhythmia rates can be variable, particularly with changing autonomic tone and antiarrhythmic drug therapy. Presentation with a monomorphic tachycardia rarely predicts subsequent freedom from VF. In fact, in clinical studies, the mortality and event rates in patients with hemodynamically “stable” VT were comparable with those in patients who had sustained a cardiac arrest.13
Device Therapies
Anti-tachycardia Pacing or Cardioversion
Tachyarrhythmia and bradyarrhythmia therapies are available in these devices. Ventricular demand pacing with or without rate response is present. Ventricular pacing output can be altered for specific situations such as anti-tachycardia pacing or post-shock bradycardia pacing. Tachyarrhythmia therapies include anti-tachycardia pacing for termination of monomorphic VT, and low- and high-energy programmable shock therapies for cardioversion of VT and defibrillation of VF.4,15 Anti-tachycardia pacing is most effective in the termination of monomorphic VT, especially with rates below 180 beats/min, although individual episodes with rates above this may respond to this therapy.1,15–17 In slow VT, the excitable gap is large; therefore anti-tachycardia pacing can easily interrupt the tachycardia. When the tachycardia is fast, the excitable gap is shorter, and anti-tachycardia pacing interruption may be more difficult. Pacing termination of rapid VT is discussed below.
Several types of anti-tachycardia pacing are available in these devices. It is not uncommon for several attempts or different algorithms to be deployed during such efforts at tachycardia termination in an individual patient. Allowances for these repetitive efforts must include the hemodynamic stability of the patient during anti-tachycardia pacing programs. These algorithms include burst pacing, which may be rate adaptive or fixed rate, and low-energy cardioversion shocks (Figure 84-5). In rate-adaptive algorithms, the pacing rate is determined by the tachycardia rate and is generally a percentage of that rate. Commonly used adaptive algorithms use adaptive rates, which can vary from 95% to 70% of the tachycardia rate, for a given number of pacing stimuli.15 Most commonly used adaptive rates for efficacy vary between 75% and 90% with 3 to 20 delivered pacing stimuli. Ramp pacing modes vary the intervals during a burst to an accelerating (positive) or decelerating (negative) ramp. Other algorithms use scanning programmed extrastimuli, usually on the basis of electrophysiology study (EPS) or ramp or burst pacing with terminal programmed scanning extrastimuli.18 In general, burst or ramp pacing, or variations thereof, provide the greatest likelihood of efficacy, especially if extensive EPS is not used for pace-termination windows and algorithms. Randomized comparative studies suggest similar efficacy for both modes.11
Clinical studies have documented a reduction in the need for defibrillator shocks with anti-tachycardia pacing from its early application to the present.18,19 The Pain Free 1 and 2 trials evaluated the use of anti-tachycardia pacing in rapid VT and its clinical impact.16,17 Anti-tachycardia pacing was performed with a predetermined adaptive pacing train at 91% of a rapid VT cycle for eight beats (Figure 84-6) to successfully terminate the episode (see Figure 84-6, A). In Pain Free 1, 32% of all VT events were in the rapid VT zone, whereas 58% were in the slow VT zone; 85% of all rapid VT events with a cycle length less than 320 ms were terminated by the initial pacing algorithm (see Figure 84-6, B), another 4% were terminated by subsequent algorithms, and only 10% of patients proceeded to shock therapy. A marked improvement was seen in quality of life measures of physical and mental functioning. These results indicate that anti-tachycardia pacing can be effective for treating fast VT and preventing painful shocks and secondary rehospitalizations. Multiple shocks for incessant VT have been shown to reduce battery longevity. Current-generation ICD devices provide anti-tachycardia pacing train delivery during charging.
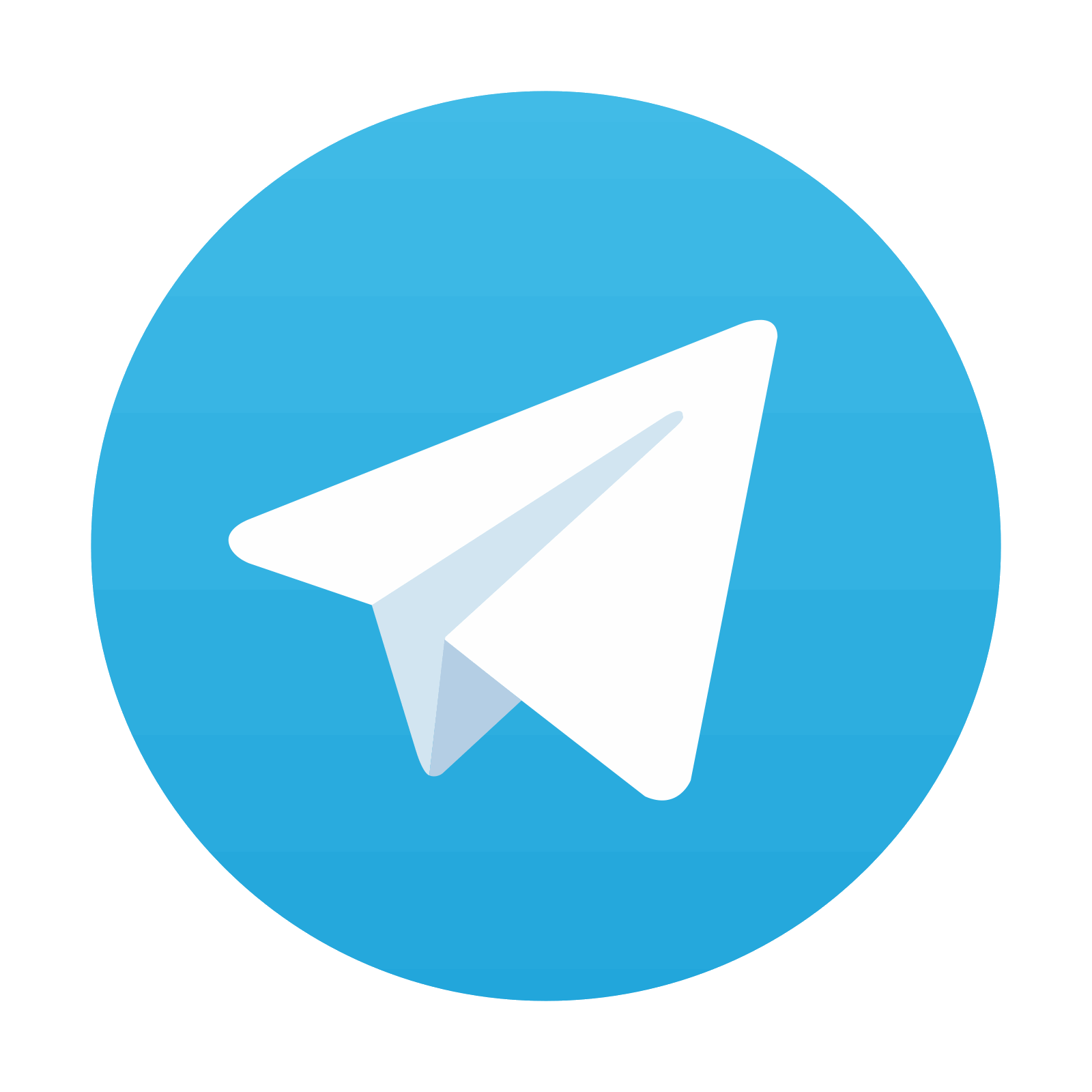
Stay updated, free articles. Join our Telegram channel
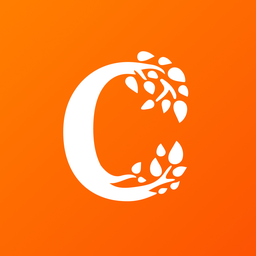
Full access? Get Clinical Tree
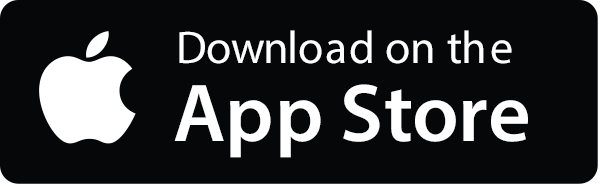
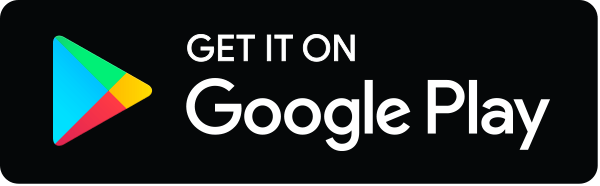
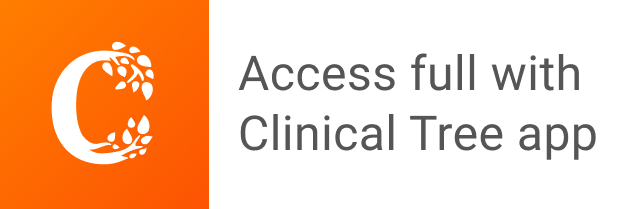