Fig. 11.1
Second Harmonic Generation images of airway and parenchymal tissues from non-diseased and diseased lungs. Second Harmonic Generation signals from fibrillar collagen I propagated in the forward (Cyan) (representing organised collagen) and backward (Magenta) (representing disorganised collagen) direction relative to the incident light (Dimensions: 246.03 × 246.03 μm). Abbreviations: COPD chronic obstructive pulmonary disease, IPF idiopathic pulmonary fibrosis , L airway lumen
Idiopathic Pulmonary Fibrosis
In IPF, remodelling of the lung tissue structure is an important contributor to the pathology [101–106]. These changes include increased deposits of fibrotic ECM proteins in the interstitium and air spaces, thickening of the airway walls and the collapse of air spaces. These tissues have increased staining for hyaluronic acid [11], the foetal form of cellular fibronectin (containing the EIIIA domain) [107] and collagen I and III [80, 108, 109], which are usually located in or around the fibrotic foci of fibroblasts that are characteristic in IPF lung tissues [106, 110, 111].
Re-cellularising the Lung : Where to Next?
The highly complex structure of the ECM and its importance in the regulation of cellular behaviours in vivo have made it a challenging structure to study [112–114]. Early studies using light and electron microscopy showed that the matrices were widespread with distinct structural arrangements that underlay many cells. Exploration of the biology of the development and functionality of the ECM has been, and continues to be, challenging due to the fact that the ECM is, by nature, crosslinked and highly insoluble [1]. The traditional two-dimensional (2D) in vitro cell culture model that is often employed in pursuit of these answers does not sufficiently reflect native biology for cost-effective and efficient translational medical research. Owing to the many limitations of in vitro studies, translating the findings from in vitro studies to in vivo and eventually human studies is an ongoing challenge (Fig. 11.2).
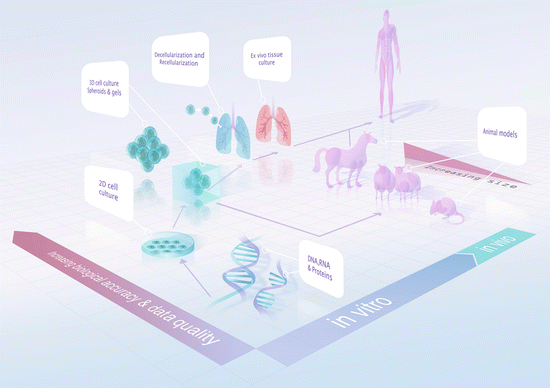
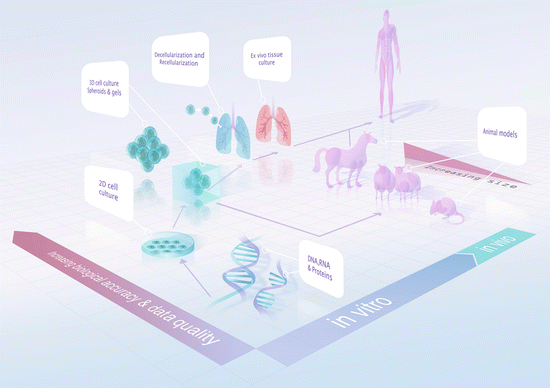
Fig. 11.2
Milestones for translational medical research
Among the many limitations of the in vitro 2D culture systems is the fact that they do not accurately represent the in vivo 3D structures. Furthermore, most in vitro culture systems utilise a plastic/glass surface—which are both non-biological, in terms of the surface composition presented to the cells, and the mechanical properties are at odds with those in the in vivo system [115]. Whilst advancements have been made in the in vitro systems utilising surfaces coated with ECM-derived materials such as collagen and fibronectin [115] or mixtures of homogenised and dissolved decellularised tissues [116], this is merely a small step towards replicating the true ECM environment in vivo.
Three-dimensional cell culture is a further advancement for the field, bringing the experimental outcome closer to biological significance. However, in vitro 3D cell culture techniques such as spheroid suspensions [117] and 3D gel cultures [115, 118–120] retain some of the limitations described for 2D systems, primarily the question of: “What are the appropriate components and structure of the ECM?” Whilst there are many studies investigating the precise combination of ECM components in various tissues using techniques such as mass spectrometry [121, 122], current in vitro 3D model systems utilise single ECM components such as collagen, fibronectin or fibrin gels [115] or combinations of a few ECM components [123]; which pale in comparison to in vivo ECM that is composed of hundreds of different components [1]. Further complications are added by the interactions between ECM components which ultimately dictate the “net biological properties” of the scaffold . As such, the construction of a biologically accurate artificial/synthetic ECM scaffold remains a major hurdle in translational research.
To counter this highly complex problem, the technique of tissue decellularisation has been developed. Decellularisation is a novel technique that produces scaffolds through the removal of cells from viable tissue (utilising a combination of chemical and mechanical treatments), thus preserving much of the original ECM content and architecture (Fig. 11.3). Scaffolds created using this technique maintain most (but not all) of their ECM components and mechanical properties, making them a suitable platform for translational studies. As with any technique, there are also limitations, the first of which is that the decellularisation process can remove some of the less adhesive components of the ECM and introduce some small structural changes. There are many different decellularisation techniques emerging, each with its own advantages and disadvantages (Table 11.1) [172] but the field is yet to agree on a standardised approach. The ideal approach would be for the protocol used for the production of the decellularised tissue to be tailored towards the type of tissue used and the study in question to ensure preservation of as many of the ECM properties as possible.
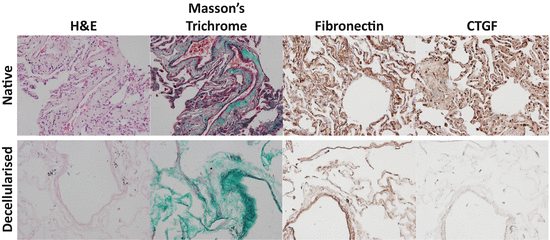
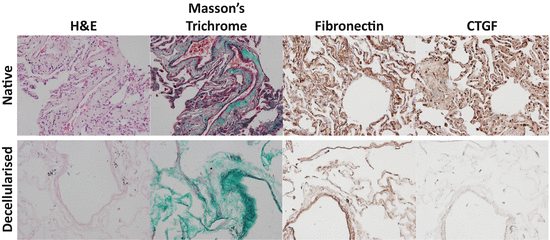
Fig. 11.3
Comparison of lung tissue components pre- and post-decellularisation. Lung tissue sections before and after decellularisation with subsequent washes in 0.1 % Triton X-100, 2 % Sodium Deoxycholate, 1 M NaCl, 30 μg/mL DNAse and sterilised with 0.018 % Peracetic acid in 4.8 % Ethanol with mild agitation were stained and imaged using a BX60 Olympus light microscope and attached DP71 camera to examine Hematoxylin and Eosin stain (blue = cell nuclei; pink = cytoplasm), Masson’s Trichrome stain (black = cell nuclei; red = muscle, cytoplasm, keratin; green = collagen), Fibronectin or CTGF using DAB detection of a specific antibody (brown). Images were captured at ×20 magnification. Abbreviations: H&E hematoxylin and eosin, CTGF connective tissue growth factor, DAB = 3,3′-diaminobenzidine
Table 11.1
The advantages and disadvantages of methods of tissue decellularisation
Agent/technique | Mode of action | Effects on ECM | References |
---|---|---|---|
Chemical agents | |||
Acids and bases | Solubilises cytoplasmic components, disrupts nucleic acids and denatures proteins | May cause damage to collagen, GAGs and growth factors | |
Hypotonic and hypertonic solutions | Cell lysis through osmotic shock with disruption to DNA–protein interaction | Effective for cell lysis but not removal of cellular residues | |
Non-ionic detergents | Disrupts interactions between DNA–protein, lipid–lipid, lipid–protein and to a lesser extent protein–protein interactions. (e.g. Triton X-100) | Mixed efficacy. Efficacy dependent on tissue, cell removal is more effective on thin tissues and some disruption of the ultrastructure and removal of GAGs may occur | |
Ionic detergents | Solubilization of cellular and nucleic membranes. Denatures proteins. (e.g. Sodium Dodecyl Sulfate (SDS), Sodium Deoxycholate and Triton X-200) | Effect varies between detergents. Effectively removes nuclear remnants and cytoplasmic proteins with tendency to disrupt the ultrastructure, removal of GAGs and may damage collagen | |
Zwitterionic detergents | Exhibits properties from both ionic and non-ionic detergents | Effective at removal of cells with mild disruption to the ultrastructure of thin tissues | |
Solvents | Alcohols and acetone lyse cells through dehydration, also solubilises and removes lipids | Effective at removal of cells from dense tissues and inactivates pyrogens but it causes proteins such as collagen to crosslink and precipitate | |
Tributyl Phosphates form stable complexes with metals and disrupts protein–protein interactions | Mixed efficacy results (dependent on tissues) but causes loss of collagen with minimal impact on mechanical properties | ||
Biological agents | |||
Enzymes | |||
Nucleases | Targets ribonucleotide and deoxy-ribonucleotide chains | Difficult to remove from tissue and can invoke immune a response | |
Trypsin | Cleaves peptide bonds | Prolonged exposure can disrupt the ECM ultrastructure and removes ECM components (e.g. collagen, laminin, fibronectin, elastin and GAGs—less effective GAG removal compared to detergents) | |
Dispase | Cleaves specific peptides (e.g. fibronectin and collagen IV) | Prolonged exposure can disrupt ECM ultrastructure and remove ECM components (e.g. fibronectin and collagen IV) | |
Chelating agents | Disrupts cell-ECM adhesion by binding metallic ions. (e.g. EDTA and EGTA) | Ineffective when used alone. Typically used in combination with enzymatic (e.g. trypsin) or other agents | |
Physical and miscellaneous agents | |||
Temperature (freeze thaw) | Intracellular ice crystals develop and disrupt cell membrane | Intracellular ice crystals can potentially disrupt or fracture the ECM | |
Direct application of force | Physically damages and bursts cells | Direct application of force can damage the ECM | |
Pressure | Pressure can burst cells and help in the removal of cellular material | Pressure can disrupt the ECM | |
Electroporation | Pulsed electrical fields can disrupt the cell membrane | Pulsed electrical fields can disrupt the ECM | |
Techniques to apply agents | |||
Perfusion | Increases chemical exposure through the tissue and helps in the removal of cellular material | Excessive pressure during perfusion can disrupt the ECM | |
Pressure gradient across tissue | Increases chemical exposure through the tissue and helps in the removal of cellular material | Pressure gradient can disrupt the ECM | |
Agitation | Can cause cell lysis and increases chemical exposure through the tissue and helps in the removal of cellular material | Excessive agitation and/or sonication can disrupt the ECM |
Re-cellularisation: The Final Goal
One of the major issues for organ transplantation programmes is the shortage of viable donor organs, followed by the immunological rejection of the implanted organ by the recipient [173–175]. The ultimate goal of tissue decellularisation is to eventually enable the re-cellularisation and repopulation of generated ECM scaffolds with recipient autologous cells to regenerate a viable tissue/organ for implantation.
The ECM scaffold has limited immunogenicity [167, 176] and through re-cellularisation of the scaffold with the recipient’s cells it should be possible to create graft tissue material for treatment purposes [141, 151, 157, 176, 177]. Whilst this process has had success in simple organ systems such as skin [172], to date it is yet to be successful in the long term for complex organs such as the lungs [153]. Current research has successfully re-cellularised the lungs from various different species with a variety of cell types (Table 11.2). Whilst re-cellularised organs can perform organ-specific functions for short periods of time [140], further research is necessary to progress and exploit this new emerging treatment possibility.
Table 11.2
Examples of successful lung tissue decellularisation and re-cellularisation
Source species | Re-cellularised cells | Reference |
---|---|---|
Murine | Mouse embryonic stem cells | |
Mouse fibroblast | [180] | |
Mouse bone marrow-derived mesenchymal stromal cells | ||
Mouse C10 lung epithelial cells | [182] | |
Mouse pulmonary alveolar epithelial cells | [130] | |
Rattus | Human small airway epithelial cells | [161] |
Human pulmonary alveolar epithelial cells | [161] | |
Human pulmonary basal epithelial cells (A549) | [156] | |
Human umbilical vein endothelial cells | ||
Mouse embryonic stem cells | [157] | |
Rat fetal lung cells | ||
Rat microvascular endothelial cells | [153] | |
Rat pulmonary alveolar epithelial cells | [153] | |
Porcine | Human lung fibroblasts | |
Human small airway epithelial cells | [183] | |
Human adipose-derived mesenchymal stem cells | [183] | |
Human bone marrow-derived mesenchymal stem cells | [151] | |
Human vascular endothelial cells (CBF12) | [151] | |
Human bronchial epithelial cells | [151] | |
Non-human primate | Primate adipose-derived mesenchymal stem cells | [184] |
Primate bone marrow-derived mesenchymal stem cells | [184] | |
Human | Human lung fibroblasts | |
Human small airway epithelial cells | ||
Human pulmonary alveolar epithelial cells | [161] | |
Human adipose-derived mesenchymal stem cells | [183] | |
Human bone marrow-derived mesenchymal stem cells | ||
Human umbilical vein endothelial cells | [161] | |
Human vascular endothelial cells (CBF12) | [151] | |
Human bronchial epithelial cells | [151] | |
Human progenitor endothelial cells | [152] |
Stem cells have been highlighted as ideal cells for re-cellularisation of the ECM scaffold as not only does the ECM scaffold possess the required structure and mechanical properties, but ECM components can also assist in guiding the cell differentiation and migration processes for optimal regeneration of the tissue/organ for implantation [5, 157]. Whether the defined populations of progenitor cells within the lung can be re-established in healthy lung niches is an exciting prospect that needs extensive investigation.
Conclusions
The ECM provides structural support and environmental cues for cells within its microenvirons. This local regulatory system enacts dedicated stem cell niches within the lung which allow for the maintenance of specialised progenitor cell populations. Greater understanding of cell signalling, preferential attachment, migration and differentiation by cells as directed by the ECM microenvironment will provide valuable insights to cell/matrix dynamics. This will lead to advances in knowledge about the roles of various ECM components and their structural interactions with cells. The field is moving towards the ultimate goal of the development of a synthetic matrix scaffold suitable for re-cellularisation [185] and eventual re-construction of a viable lung for transplant purposes.
References
1.
Hynes RO, Naba A (2012) Overview of the matrisome—an inventory of extracellular matrix constituents and functions. Cold Spring Harb Perspect Biol 4(1):a004903PubMedCentralPubMed
2.
Roberts CR, Walker DC, Schellenberg RR (2002) Extracellular matrix. Clin Allergy Immunol 16:143–178PubMed
3.
Frantz C, Stewart KM, Weaver VM (2010) The extracellular matrix at a glance. J Cell Sci 123(Pt 24):4195–4200PubMedCentralPubMed
4.
Tran T, Halayko AJ (2007) Extracellular matrix and airway smooth muscle interactions: a target for modulating airway wall remodelling and hyperresponsiveness? Can J Physiol Pharmacol 85(7):666–671PubMed
5.
Raghavan S, Bitar KN (2014) The influence of extracellular matrix composition on the differentiation of neuronal subtypes in tissue engineered innervated intestinal smooth muscle sheets. Biomaterials 35(26):7429–7440PubMedCentralPubMed
6.
Pelosi P, Rocco PRM, Negrini D, Passi A (2007) The extracellular matrix of the lung and its role in edema formation. An Acad Bras Cienc 79(2):285–297PubMed
7.
Marastoni S, Ligresti G, Lorenzon E, Colombatti A, Mongiat M (2008) Extracellular matrix: a matter of life and death. Connect Tissue Res 49(3):203–206PubMed
8.
Negrini D, Passi A, de Luca G, Miserocchi G (1998) Role of matrix macromolecules in controlling lung fluid balance: transition from a dry tissue to edema. In: Matalon S, Sznajder JI (eds) Acute respiratory distress syndrome: cellular and molecular mechanisms and clinical management, vol 297. Plenum, New York, pp 65–68
9.
Suki B, Ito S, Stamenovic D, Lutchen KR, Ingenito EP (2005) Biomechanics of the lung parenchyma: critical roles of collagen and mechanical forces. J Appl Physiol 98(5):1892–1899PubMed
10.
Negrini D, Tenstad O, Passi A, Wiig H (2006) Differential degradation of matrix proteoglycans and edema development in rabbit lung. Am J Physiol Lung Cell Mol Physiol 290(3):L470–L477PubMed
11.
Papakonstantinou E, Karakiulakis G (2009) The ‘sweet’ and ‘bitter’ involvement of glycosaminoglycans in lung diseases: pharmacotherapeutic relevance. Br J Pharmacol 157(7):1111–1127PubMedCentralPubMed
12.
Dunsmore SE (2008) Treatment of COPD: a matrix perspective. Int J Chron Obstruct Pulmon Dis 3(1):113–122PubMedCentralPubMed
13.
Roberts CR, Rains JK, Pare PD, Walker DC, Wiggs B, Bert JL (1998) Ultrastructure and tensile properties of human tracheal cartilage. J Biomech 31(1):81–86PubMed
14.
Kim HY, Nelson CM (2012) Extracellular matrix and cytoskeletal dynamics during branching morphogenesis. Organogenesis 8(2):56–64PubMedCentralPubMed
15.
Kumar ME, Bogard PE, Espinoza FH, Menke DB, Kingsley DM, Krasnow MA (2014) Mesenchymal cells. Defining a mesenchymal progenitor niche at single-cell resolution. Science 346(6211):1258810PubMedCentralPubMed
16.
Matsuo I, Kimura-Yoshida C (2014) Extracellular distribution of diffusible growth factors controlled by heparan sulfate proteoglycans during mammalian embryogenesis. Philos Trans R Soc Lond B Biol Sci 369(1657)
17.
Hynes RO (2009) The extracellular matrix: not just pretty fibrils. Science 326(5957):1216–1219PubMedCentralPubMed
18.
McGowan SE (1992) Extracellular matrix and the regulation of lung development and repair. FASEB J 6(11):2895–2904PubMed
19.
Shannon JM (1994) Induction of alveolar type II cell differentiation in fetal tracheal epithelium by grafted distal lung mesenchyme. Dev Biol 166(2):600–614PubMed
20.
Antunes MA et al (2009) Different strains of mice present distinct lung tissue mechanics and extracellular matrix composition in a model of chronic allergic asthma. Respir Physiol Neurobiol 165(2–3):202–207PubMed
21.
Freyer AM, Johnson SR, Hall IP (2001) Effects of growth factors and extracellular matrix on survival of human airway smooth muscle cells. Am J Respir Cell Mol Biol 25(5):569–576PubMed
22.
Hirst SJ, Twort CH, Lee TH (2000) Differential effects of extracellular matrix proteins on human airway smooth muscle cell proliferation and phenotype. Am J Respir Cell Mol Biol 23(3):335–344PubMed
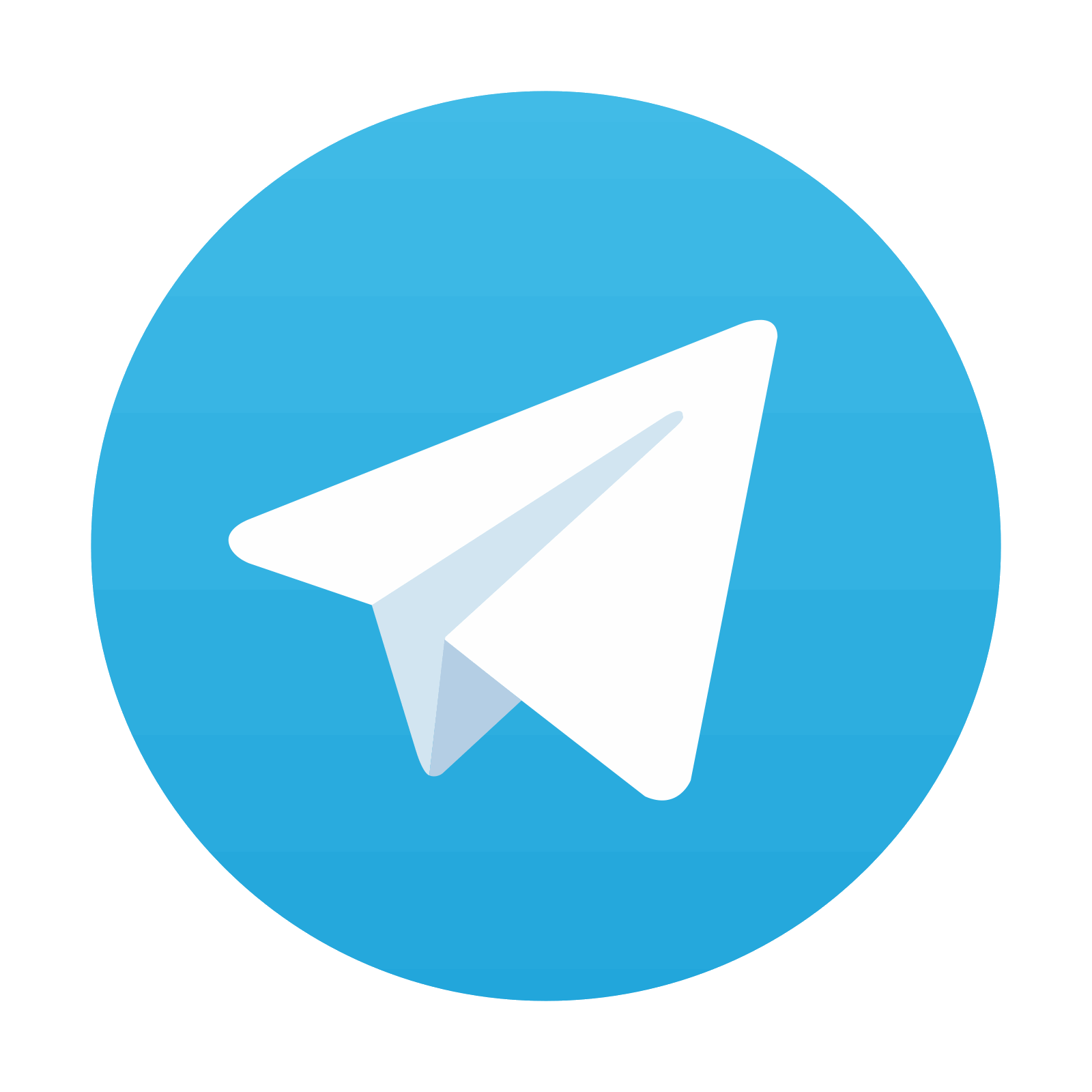
Stay updated, free articles. Join our Telegram channel
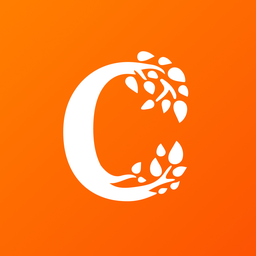
Full access? Get Clinical Tree
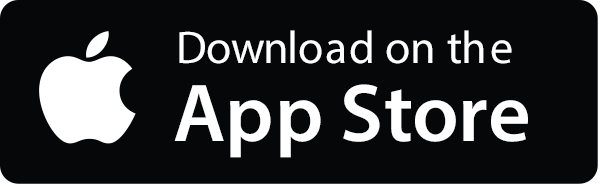
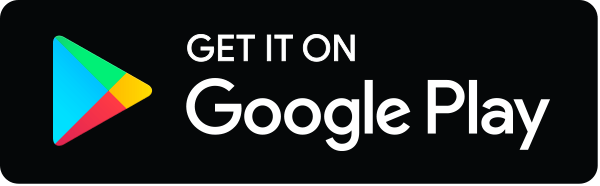