Key Points
- •
Early lesions of atherosclerosis begin in childhood and are related to antecedent CVD risk factors. Environmental factors such as diet, obesity, and exercise and certain inherited dyslipidemias influence the progression of such lesions. Detection of youth at risk for atherosclerosis includes an integrated evaluation of these predisposing factors.
- •
Treatment starts with weight control, exercise, and a diet low in saturated fat, trans -fat, and cholesterol, supplemented with water-soluble fiber, plant stanols, or plant sterols.
- •
Drug therapy, especially with HMG-CoA reductase inhibitors or bile acid sequestrants, can be considered in those with a positive family history of premature CVD and LDL-C ≥160 mg/dL, after a period of dietary and hygienic measures. Candidates for drug therapy often include those with familial hypercholesterolemia, familial combined hyperlipidemia, the metabolic syndrome, polycystic ovarian syndrome, type 1 or 2 diabetes, and the nephrotic syndrome.
- •
Dietary and drug therapy appears to be safe and efficacious.
- •
Early identification and treatment of children with CVD risk factors and dyslipidemia are likely to retard the atherosclerotic process.
- •
Optimal identification and treatment of high-risk youth require an integrated universal screening, evaluation, treatment, and follow-up program.
Disorders of lipid and lipoprotein metabolism are characterized by abnormalities of the major lipoprotein classes, chylomicrons, very-low-density lipoproteins (VLDL), low-density lipoproteins (LDL), and high-density lipoproteins (HDL). Dyslipidemia can result from the expression of a mutation in a single gene that plays a significant role in lipoprotein metabolism, reflect the effects of multiple genes, or be caused by environmental influences such as excessive dietary intake of fat and calories and limited physical activity, particularly in association with overweight or obesity.
The major clinical complication of dyslipidemia is a predisposition to atherosclerosis starting early in life and leading to cardiovascular disease (CVD) in adulthood. Multiple trials in adults have demonstrated that reduction in the plasma levels of LDL results in the decreased prevalence of coronary artery disease (CAD) and stroke. Lowering of LDL also produces a decrease in the angiographic progression of CAD and even modest regression in some cases. In children with familial hypercholesterolemia, a decrease in LDL reduces carotid intima-media thickness (CIMT) and lipid screening recommendations have recently been updated by the American Academy of Pediatrics.
This chapter presents a theoretical and practical approach to the diagnosis and management of dyslipidemia in children and adolescents, in the hope of preventing or retarding the progression of atherosclerosis early in life.
Background
A number of studies strongly support the notion that atherosclerosis and CVD risk factors originate in childhood and adolescence and that treatment should begin early in life. Several longitudinal pathologic studies found that early atherosclerotic lesions of fatty streaks and fibrous plaques in children, adolescents, and young adults who died of accidental deaths are significantly related to higher antecedent levels of total cholesterol (TC) and LDL-cholesterol (LDL-C), lower levels of HDL-cholesterol (HDL-C), and other CVD risk factors, such as higher blood pressure, cigarette smoking, and obesity.
Major prospective population studies, including the Muscatine Study, the Bogalusa Heart Study, the Coronary Artery Risk Development in Young Adults (CARDIA) study, the Special Turku Coronary Risk Factor Intervention Project (STRIP), and the Cardiovascular Risk in Young Finns Study, demonstrated that CVD risk factors in children and adolescents, particularly LDL-C and obesity, predicted subclinical manifestations of atherosclerosis in young adults, as estimated by coronary artery calcium, CIMT, or brachial flow–mediated dilation. With respect to clinical manifestations of atherosclerosis, medical students at Johns Hopkins who had a TC level >207 mg/dL had five times the risk for development of CVD 40 years later than did those students who had a TC level <172 mg/dL.
Studies in high-risk youth, selected by virtue of CVD in one parent, demonstrated the familial aggregation of dyslipidemia in such children. For example, half of the young progeny of men with premature CVD before 50 years of age had one of seven dyslipidemic profiles: elevated LDL-C alone (type IIa) or combined with high triglycerides (type IIb); elevated triglycerides alone (type IV); low HDL-C alone (hypoalphalipoproteinemia); and type IIa, type IIb, or type IV also accompanied by low HDL-C. Elevated levels of apolipoprotein B, in the presence of normal LDL-C (hyperapobetalipoproteinemia), were prevalent in young offspring of adults with premature CVD and hyperapobetalipoproteinemia. The levels of apolipoprotein B (apo B) and apolipoprotein A-I (apo A-I), the major apolipoproteins of LDL and HDL, respectively, and the ratio of apo B to apo A-I in young offspring from Bogalusa were stronger predictors of premature CAD in their parents than the levels of either LDL-C or HDL-C.
Inherited lipoprotein disorders that often present in youth at high risk for future CVD include familial hypercholesterolemia (FH), caused by a defect in the LDL receptor (LDLR), and familial combined hyperlipidemia (FCHL) and its metabolic cousin, hyperapobetalipoproteinemia, both prototypes for overproduction of VLDL in the liver. Increased synthesis and secretion of VLDL are often driven by an increased flux of free fatty acids (FFA) from the adipose tissue to the liver, a metabolic abnormality usually accompanied by insulin resistance and the dyslipidemic triad of elevated triglycerides, increased small, dense LDL particles (sLDL-P), and low HDL-C.
In addition, secondary accelerated atherosclerosis requiring aggressive lipid management can be encountered in certain clinical settings early in life, such as in children and adolescents with diabetes mellitus, end-stage renal disease on hemodialysis, or nephrotic syndrome; after renal or heart transplantation; in HIV-positive patients receiving protease inhibitors; in childhood cancer survivors; or in children and adolescents receiving chronic antipsychotic treatment.
Lipid and Lipoprotein Classification
Cholesterol, triglycerides (TG), phospholipids (PL), and cholesteryl esters (CE) are major lipid classes that are essential components found in dietary fat, plasma lipoproteins, and cells. Lipids are hydrophobic and do not circulate freely in plasma but rather as part of lipid-protein macromolecular complexes called lipoproteins. Plasma lipoproteins are generally spherical particles consisting of a core that contains nonpolar lipids, mostly TG and CE, surrounded by a surface coating consisting of proteins (apolipoproteins), and polar lipids, such as PL and unesterified (free) cholesterol (FC). Lipoproteins function as transport vehicles for water-insoluble lipids and carry them to their sites of metabolism or deposition.
Plasma lipoproteins have been classified by their density and electrophoretic mobility into chylomicrons, VLDL (density <1.006 g/mL) or pre-beta (pre-β) lipoproteins, LDL (density 1.019-1.063 g/mL) or beta (β) lipoproteins, and HDL (density 1.063-1.21 g/mL) or alpha (α) lipoproteins ( Table 12-1 ). Intermediate-density lipoproteins (IDL; density 1.006-1.019 g/mL; also called VLDL remnants) are produced after the hydrolysis of the TG on VLDL. Electrophoresis permits the separation of the plasma lipoproteins on the basis of differences in size and electrophoretic charge. After electrophoresis, chylomicrons remain at the origin, and VLDL, LDL, and HDL migrate in the same positions as pre-β–, β-, and α-globulins, respectively. IDL migrate to a position between the pre-β– and β-globulins. The hydrated density of the lipoproteins is related to their chemical composition and the relative content of lipid and apolipoprotein.
Chylomicrons | Very-Low-Density Lipoproteins | Low-Density Lipoproteins | High-Density Lipoproteins | |
---|---|---|---|---|
Hydrated density (g/mL) | <0.95 | 0.95-1.006 | 1.019-1.063 | 1.063-1.21 |
Electrophoretic migration | Origin | Pre-β | β | α |
Molecular weight | 50-1000 × 10 6 | 10-80 × 10 6 | 2.3 × 10 6 | 1.8-3.6 × 10 5 |
Average composition (%) | ||||
Cholesterol * Triglyceride Phospholipid Protein | 3 90 6 1 | 22 55 15 8 | 50 5 25 20 | 20 5 25 50 |
Major apolipoproteins | Apo B-48 Apo A-I, IV | Apo B-100 Apo C-I, II, III Apo E | Apo B-100 | Apo A-I, II Apo C-I, II, III Apo E |
Origin | Intestine | Liver | Product of VLDL catabolism | Liver, intestine |
Function | Transport intestinal triglycerides and cholesterol | Transport hepatic triglycerides and cholesterol | Provide cholesterol to cells | Reverse cholesterol transport |
* Includes the mass of cholesteryl ester and esterified cholesterol.
Chylomicrons contain 99% lipid, mostly TG (see Table 12-1 ). After plasma is stored overnight in a tube, these large particles (80 to 500 nm) rise to the top, where they appear as a creamy layer. VLDL contain 90% lipid, the majority being TG, with lesser amounts of cholesterol. When they are present in plasma in increased amounts, VLDL particles are large enough (30 to 80 nm) to impart a cloudy or turbid appearance to plasma. LDL are the major carriers of cholesterol in plasma, and about 50% of their weight is CE and FC. LDL are a heterogeneous group whose size and density vary according to the core content of CE. HDL are often composed of about 50% apolipoprotein and about equal amounts of cholesterol and PL.
Apolipoproteins
Plasma lipoprotein classes are associated with a number of apolipoproteins ( Table 12-2 ). The apolipoproteins have a number of functions; they solubilize lipids in aqueous plasma, permit secretion of chylomicrons and VLDL from intestine and liver, and serve as cofactors for important enzymes in lipoprotein metabolism, and they are responsible for the binding of lipoproteins to specific receptors. The characteristics of the 11 major apolipoproteins and their functions are summarized in Table 12-2 . The nucleotide sequences of cDNA for these apolipoproteins have been determined.
Apolipoprotein | Molecular Weight | Chromosomal Location | Function |
---|---|---|---|
Apo A-I | 29,016 | 11q23 | Cofactor LCAT; facilitates both the transfer of cell cholesterol by ABCA-1 to nascent HDL and the delivery of CE and FC on HDL to liver through SR-B1 |
Apo A-II | 17,414 | 1q21-23 | Inhibits TG hydrolysis by HL and VLDL |
Apo A-IV | 44,465 | 11q23 | Activates LCAT, promotes formation of chylomicrons |
Apo A-V | 39,000 | 11q23 | Stimulates proteoglycan-bound LPL |
Apo B-100 | 512,723 | 2p24-p23 | Secretion of VLDL from liver; binding ligand to LDLR |
Apo B-48 | 240,800 | 2p24-p23 | Secretion of chylomicrons from intestine |
Apo C-I | 6630 | 19q13.2 | Inhibits apo E binding to LDLR; stimulates LCAT; inhibits CETP and SR-B1 |
Apo C-II | 8900 | 19q13.2 | Cofactor LPL |
Apo C-III | 8800 | 11q23 | Noncompetitive inhibitor of LPL; inhibits binding of apo E on TG-rich lipoproteins to LDLR |
Apo D | 19,000 | 3q26.2 | Promotes reverse cholesterol transport |
Apo E | 34,145 | 19q13.2 | Binding ligand for LRP on chylomicron remnants and VLDL and IDL for LDLR |
Lipid and Lipoprotein Metabolism
The transport of plasma lipids by lipoproteins may be divided into an exogenous (dietary) pathway, an endogenous (hepatic) pathway, and reverse cholesterol transport ( Fig. 12-1 ).

Exogenous Lipid Transport
Most of the lipid in the diet is present as neutral fat or TG (75 to 150 g/day). Dietary cholesterol intake is usually about 300 to 400 mg/day but varies from 100 to 600 mg/day. In addition to dietary cholesterol, about 1100 mg of biliary cholesterol is secreted each day from the liver into the intestine (see Fig. 12-1 ). In the small intestine, lipids are emulsified by bile salts and hydrolyzed by pancreatic lipases. TG are broken down into FFA and 2-monoglycerides; CE are hydrolyzed into FC and FFA. These lipid components are then absorbed by the intestinal cells. Bile acids are reabsorbed by the intestinal bile acid transporter (IBAT) for return to the liver through the enterohepatic circulation (see Fig. 12-1 ).
The absorption of cholesterol occurs in the jejunum, through the high-affinity uptake of dietary and biliary cholesterol by the Niemann-Pick C1-like 1 (NPC1L1) protein (see Fig. 12-1 ). Normally, about half the dietary and biliary cholesterol is absorbed daily. Excessive cholesterol absorption is prevented by the ATP-binding cassette, subfamily G (ABCG5/ABCG8) transporters, which act together to pump excess cholesterol and plant sterols from the intestine back into the lumen for fecal excretion (see Fig. 12-1 ).
Inside intestinal cells, monoglyceride is re-esterified into TG and cholesterol is esterified by acyl:cholesterol acyltransferase (ACAT). Both TG and CE are packaged into chylomicrons, along with apo A-I, apo A-IV, and apo B-48. Chylomicrons are secreted into the thoracic duct, from which they enter the peripheral circulation, where they acquire apo C-II and apo E from HDL. Chylomicrons are too large to cross the endothelial barrier, and apo C-II, a cofactor for lipoprotein lipase (LPL), facilitates the hydrolysis of TG near the endothelial lining of blood vessels.
FFA that are released from the hydrolysis of TG are taken up by muscle cells for energy use or by adipose cells for re-esterification into TG and storage. As a result, a chylomicron remnant is produced that is relatively enriched in CE and apo E. This remnant is rapidly taken up by the liver by a process that involves an initial sequestration of remnant particles on hepatic cell surface proteoglycans. Receptor-mediated endocytosis of remnants follows through the interaction of apo E either with the low-density receptor–like protein (LRP, also called the chylomicron remnant receptor) or with the LDLR, both on the surface of hepatic parenchymal cells (see Fig. 12-1 ).
The uptake of dietary and biliary cholesterol is part of a process that regulates the pool of hepatic cholesterol by downregulation of the LDLR and by inhibition of the rate-limiting enzyme of cholesterol biosynthesis, hydroxymethylglutaryl–coenzyme A (HMG-CoA) reductase (see also later). Hepatic cholesterol can be secreted into bile unchanged, converted into bile acids by 7α-hydroxylase (CYP7A1), or used for lipoprotein synthesis (see Fig. 12-1 ).
Endogenous Lipid Transport
In the fasting state, most TG in plasma are carried by VLDL. TG are synthesized in the liver and packaged into VLDL with other lipids and apolipoproteins (see Table 12-1 ), primarily apo B-100, apo E, apo C-I, apo C-II, and apo C-III, and secreted into plasma. The TG in VLDL are subsequently hydrolyzed by LPL, and its cofactor apo C-II, to produce VLDL remnants or IDL. TG can be transferred from VLDL and IDL to HDL and LDL in exchange for CE by the cholesteryl ester transfer protein (CETP; see Fig. 12-1 ). Compared with VLDL, IDL are relatively enriched in CE and depleted of TG. Some IDL are taken up directly by the liver, but others are hydrolyzed by hepatic lipase to produce LDL, the final end product of VLDL metabolism (see Fig. 12-1 ).
The apo B-100 component of the CE-rich LDL binds with high affinity to the LDLR, in either liver or extrahepatic cells (see Fig. 12-1 ). Bound LDL is internalized by absorptive endocytosis. In lysosomes, apo B-100 is broken down into amino acids, CE hydrolyzed, and FC released. Cholesterol mediates the proteolytic release of a transcription factor, the sterol regulatory element–binding protein (SREBP), from the endoplasmic reticulum. This effect occurs through the SREBP cleavage-activating protein (SCAP) that is both a sensor of sterols and an escort of SREBP from the endoplasmic reticulum to the Golgi.
The family of transcription factors SREBP regulates the synthesis of cholesterol and fatty acids. SREBP-2 mainly regulates cholesterol synthesis. When hepatocytes are depleted of cholesterol, SCAP transports SREBP from the endoplasmic reticulum to the Golgi, where two proteases, Site-1 protease and Site-2 protease, act in sequence to release the NH 2 -terminal domain of SREBP from the membrane. The NH 2 -terminal bHLH-Zip domain of SREBP enters the nucleus and binds to a sterol response element in the promoter area of the LDLR and HMG-CoA reductase genes, increasing their transcription. As the cholesterol content of the hepatocyte increases, the SREBP/SCAP complex is not incorporated into the endoplasmic reticulum, SREBP cannot reach the Golgi, and the NH 2 -terminal domain of SREBP cannot be released from the membrane for transport into the nucleus, and the transcription of the LDLR and HMG-CoA reductase genes decreases.
This pathway has important clinical implications. For example, excess dietary and biliary cholesterol leads to downregulation of the LDLR and HMG-CoA reductase and an increase in LDL-C. Dietary saturated fat content has a greater effect on LDL-C than dietary cholesterol does. When cholesterol is re-esterified by ACAT, SCAP senses a decrease in hepatic cholesterol, leading to upregulation of the LDLR and HMG-CoA reductase genes by SREBP. However, the preferred substrate for ACAT is oleic acid. Thus, excess saturated fatty acids decrease ACAT activity and thereby increase FC, which inhibits the proteolysis and release of SREBP; this downregulates the LDLR and HMG-CoA reductase genes, followed by an increase in LDL-C.
Decrease of dietary cholesterol and saturated fatty acids or of the hepatic cholesterol content with pharmacologic agents, such as a cholesterol absorption inhibitor or a bile acid sequestrant (see Fig. 12-1 ), leads to upregulation of LDLR and HMG-CoA reductase genes and lowers LDL-C. HMG-CoA reductase inhibitors (statins) also reduce hepatic cholesterol content, leading to upregulation of LDLR but without the concomitant increase in HMG-CoA reductase activity (see Fig. 12-1 ). The concomitant use of a statin with either a cholesterol absorption inhibitor or bile acid sequestrant provides a complementary reduction in hepatic cholesterol and consequently LDL-C.
When plasma LDL-C exceeds 100 mg/dL, the capacity to process LDL through the LDLR pathway is exceeded. Increased numbers of LDL particles cross the endothelial barrier. LDL is trapped in the vascular wall by proteoglycans and then modified by either oxidation or glycation. Such modified LDL binds to the scavenger receptors CD36 (macrophage scavenger receptor B) and SR-A (scavenger receptor A) and enters macrophages by a low-affinity, LDLR-independent mechanism (see Fig. 12-1 ).
This pathway is not subject to feedback inhibition of LDLR synthesis by LDL-derived cholesterol. Thus, LDL continues to be taken up in an unregulated fashion, leading to excess deposition of FC and CE in macrophages (see Fig. 12-1 ). Dyslipidemias that favor an increased uptake of LDL through the scavenger pathway promote the production of foam cells and their associated atherosclerosis and xanthomas.
Pathways of HDL Metabolism and Reverse Cholesterol Transport
Synthesis of HDL
Nascent HDL
Apo A-1 is released as a lipid-free protein from the intestine and liver. Apo A-1 interacts with the ATP-binding cassette transporter 1 (ABCA1) on the basolateral membranes of hepatocytes, enterocytes, and macrophages, acquiring PL and FC to form a more stable nascent HDL particle.
Formation of Larger, Mature HDL Particle
The transition of HDL particles from the disc-shaped nascent HDL to the spherical “mature” HDL requires the esterification of cholesterol to create a hydrophobic core. Lecithin-cholesterol acyltransferase (LCAT) associates with HDL and catalyzes the transfer of FFA from lecithin to FC, forming CE. Apo A-I is a cofactor for this reaction (see Fig. 12-1 ). CE formed by this reaction constitute the neutral lipid core of mature spherical HDL 3 ; further activity of LCAT provides additional CE for the core of HDL 3 , forming the larger HDL 2 particles (see Fig. 12-1 ).
Subsequent addition of cellular cholesterol to the HDL particle occurs in a number of tissues through the action of another ATP-binding cassette transporter 1 (ABCG1) and the scavenger receptor class B1 (SR-B1), molecules that prefer larger HDL as acceptors. In addition to acquiring lipids from liver and intestine, HDL also acquires lipids from chylomicrons and VLDL in the course of hydrolysis of TG by LPL. During this process, apo A-I is transferred from chylomicrons to HDL, and apo C-II and apo E on HDL are transferred to the TG-rich lipoproteins. The TG-rich lipoproteins shed excess PL and cholesterol that are transported to HDL by the phospholipid transfer protein (PLTP).
Transfer of Lipid Between HDL and the Apo B–Containing Lipoproteins
CE are transferred by CETP from the core of spherical HDL to the apo B–containing lipoproteins in exchange for TG (see Fig. 12-1 ). This process depletes CE but enriches TG in HDL particles and has important implications for HDL metabolism. For example, if the TG in HDL are hydrolyzed by hepatic lipase, a smaller HDL particle is produced that is more avidly removed from plasma by cubilin in the kidney. PLTP is structurally similar to CETP and mediates the transfer of unsaturated fatty acids on PL from the apo B–containing lipoproteins to HDL, contributing to the acquisition of PL by HDL.
Reverse Cholesterol Transport
The CE on the spherical HDL can be transported back to liver by two mechanisms. CE are transferred from HDL to the apo B–containing lipoproteins by CETP, from which they are taken up by the LDLR (see Fig. 12-1 ). CE may also be delivered directly to the liver through SR-B1 (also called the HDL receptor). These reactions are part of a process called reverse cholesterol transport.
Once the CE is delivered to the liver and hydrolyzed into FC and FFA, the FC can be excreted directly into bile or converted into bile acids by 7α-hydroxylase. Both these pathways result in delivery of sterol from peripheral tissues and plasma into intestinal cells, promoting the excretion of sterols into the stool. Reverse cholesterol transport has been postulated to explain, at least in part, the protective effect that HDL and apo A-I have against the development of atherosclerosis. Conversely, factors that impede this process, such as a dysfunctional HDL, appear to promote atherosclerosis.
Lipid Changes Throughout Childhood and Adolescence
Clearly, the plasma levels of lipids and lipoproteins result from the influence of a variety of metabolic, genetic, and environmental factors. In the general population, these levels usually follow a gaussian distribution, often skewed toward higher levels. Percentiles are therefore used to describe such data, with the 50th percentile being the median or average, the 75th to 95th percentiles borderline elevated, and >95th percentile high or elevated. To define borderline low levels, the 25th to 5th percentiles are used, and <5th percentile is a low level.
Data from the Lipid Research Clinics have often been used to define such percentiles in youth, including the National Cholesterol Education Program (NCEP) Expert Panel on Blood Cholesterol Levels in Childhood in 1992. Similar data are available from such studies as Bogalusa. There are also data from the National Health and Nutrition Examination Survey (NHANES) on apo B and apo A-I levels and more recent data on TC and LDL-C levels.
Human plasma cholesterol levels are lowest during intrauterine life. At birth, the mean (1 SD) plasma levels in normal infants have been reported as follows: TC, 74 mg/dL ; LDL-C, 31 mg/dL ; HDL-C, 37 mg/dL ; and TG, 37 mg/dL. The plasma TC and LDL-C levels increase rapidly in the first weeks of life. The kind and source of milk in the infant’s diet can markedly influence cholesterol levels. After 2 years of age, the levels of the lipids and lipoproteins become constant up to adolescence. During adolescence, there is a 10% to 20% fall in TC and LDL-C, boys and girls being similarly affected. As well, in the second decade, the TG are higher than they are in the first decade. HDL-C decreases about 10 mg/dL in boys and stays relatively constant in girls. This information has important implications for the timing of lipid and lipoprotein screening and the cut points used because lipid concentrations are age and maturation dependent and appear influenced by gender and race as well, albeit to a small degree.
With use of data combined from three major population-based prospective cohort studies, TC, LDL-C, HDL-C, and TG variables in childhood and adolescence were classified on the basis of NCEP cut points ( Table 12-3 ), age and gender (not race specific), and NHANES cut points and compared for their ability to predict abnormal levels in adulthood. NCEP cut points (compared with NHANES cut points) were more strongly predictive of high TC, LDL-C, and TG levels in adults but less predictive of low HDL-C.
Category | Acceptable | Borderline | High † |
---|---|---|---|
Total cholesterol | <170 | 170-199 | ≥200 |
LDL-cholesterol | <110 | 110-129 | ≥130 |
Non–HDL-cholesterol | <123 | 123-143 | ≥144 |
Apolipoprotein B | <90 | 90-109 | ≥110 |
Triglycerides | |||
0-9 years | <75 | 75-99 | ≥100 |
10-19 years | <90 | 90-129 | ≥130 |
Category | Acceptable | Borderline | Low † |
---|---|---|---|
HDL-cholesterol | >45 | 35-45 | <35 |
Apolipoprotein A-I | >120 | 110-120 | <110 |
* Values for plasma lipid and lipoprotein levels are from the National Cholesterol Education Program (NCEP) Expert Panel on Cholesterol Levels in Children. Non–HDL-cholesterol values from Bogalusa are equivalent to NCEP Pediatric Panel cut points for LDL-cholesterol. Values for plasma apolipoprotein B and A-I are from the National Health and Nutrition Examination Survey III (NHANES III).
† The cut points for a high or low value represent approximately the 95th and 5th percentiles, respectively.
The continued use of the current NCEP cut points for TC, LDL-C, and TG levels in adolescents appears indicated. Thus, multiple cut points by single age and gender do not appear warranted. NHANES cut points (compared with NCEP cut points) were more strongly predictive of HDL-C in adults. The cut point for HDL-C might be revised upward, perhaps to 40 mg/dL, to improve the sensitivity of this measurement to predict low HDL-C in adults and to make the cut point congruent with that used in the grown-ups.
Definition of Dyslipidemia
Cut points to define elevated TC, LDL-C, apo B, non–HDL-C, and TG and low HDL-C and apo A-I in children and adolescents are found in Table 12-3 . Dyslipidemia is present if one or more of these lipid, lipoprotein, or apolipoprotein factors are abnormal.
Significant percentages of children and adolescents have elevated concentrations of lipids and lipoproteins. In the Child and Adolescent Trial for Cardiovascular Health (CATCH), 13% of children in the fourth grade had TC ≥200 mg/dL, with 16% of girls and 11% of boys being affected. In the 1988-1994 NHANES, approximately 10% of adolescents had TC ≥200 mg/dL. Using data combined from the 1988-2002 NHANES surveys, Jolliffe and Janssen found that the 95th percentile cut points for LDL-C had increased, indicating a shift in the distribution toward higher LDL-C values.
An important epidemiologic aspect of cardiovascular risk in childhood is the tracking of lipid and lipoprotein concentrations over time. Tracking indicates the likelihood that children will maintain their percentile ranking over time and has been demonstrated in a number of studies. In the Muscatine Study, 75% of school-aged children who had TC >90th percentile at baseline had TC >200 mg/dL in their early 20s. In the Bogalusa Heart Study, 70% of children with elevated cholesterol continued to have increased cholesterol levels in young adulthood. The Muscatine investigators also found that onset of obesity in adolescence and young adulthood, cigarette smoking, and use of oral contraceptives may have deleterious effects on adult concentrations of lipids and lipoproteins.
Screening for Dyslipidemia in Youth
Two major approaches have been used to detect dyslipidemia in youth, namely, screening in the general population and in a selected population. The extensive literature related to these two screening approaches has been reviewed in detail. Lipid screening recommendations have recently been updated by the American Academy of Pediatrics. Traditionally, screening for dyslipidemias in high-risk children has been recommended because of multiple CVD risk factors and a family history of premature CVD or the presence of hypercholesterolemia. LDL-C has been the main focus of diagnosis and treatment.
Less attention has been paid to HDL-C and TG levels. With obesity, type 2 diabetes, and the metabolic syndrome increasing in the younger population, the focus of screening is likely to be expanded to include other factors, such as obesity, low HDL-C, non–HDL-C (TC minus HDL-C), elevated TG, elevated apo B (reflecting increased numbers of LDL particles), glucose intolerance and insulin resistance, and higher blood pressure levels. Both the current and evolving concepts in screening for dyslipidemia in youth are discussed.
Selective Screening
The individualized approach identifies and treats children and adolescents at risk of having high cholesterol levels. Expanded recommendations of the NCEP Expert Panel on Blood Cholesterol Levels in Children and Adolescents from 1992 include performance of selective screening if one of the following conditions is present:
- 1
A lipoprotein profile in youth whose parents, grandparents, or siblings required coronary artery bypass graft surgery or percutaneous coronary intervention before the age of 55 years because of premature CAD.
- 2
A lipoprotein profile in those with a family history of myocardial infarction, angina pectoris, peripheral or cerebral vascular disease, or sudden death before the age of 55 years.
- 3
A TC measurement in those whose parents have high TC levels (>240 mg/dL). This recommendation might be usefully expanded to a lipoprotein profile in offspring of parents who have any genetically transmissible disorder of lipid metabolism associated with increased atherosclerosis risk, involving elevated LDL-C, non–HDL-C, apo B, TG, low HDL-C, and perhaps Lp(a).
- 4
A lipoprotein profile if the parental or grandparental family history is not known and the patient has two or more other risk factors for CAD including obesity (body mass index ≥30), hypertension, cigarette smoking, low HDL-C, physical inactivity, and diabetes mellitus. This recommendation might be usefully expanded to a lipoprotein profile if either obesity (body mass index ≥95th percentile) or overweight (body mass index 85th to 94th percentile) is present, regardless of the presence of other non-lipid CVD risk factors, to identify children and adolescents with abdominal obesity who do not meet the body mass index criterion.
The NCEP Expert Panel on Blood Cholesterol Levels in Children and Adolescents recommendations from 1992 have been modified in the most recent American Academy of Pediatrics statement, which recommends screening of children with a family history of premature CVD or high cholesterol or those for whom a family history is unknown or those with other risk factors for CVD (e.g., obesity, hypertension, smoking). An optimal program would identify individuals at greatest risk for CVD in adulthood; however, there is currently no clinically applicable noninvasive screening tool available to assess this in children without familial hypercholesterolemia. Whereas the current targeted approach based on family history assumes that this information is known, with adult family members information is often not available.
Universal Screening
Universal lipid screening of all children is controversial. There are a number of advantages and disadvantages of universal screening. What are some of the arguments in favor of universal screening? First, current screening recommendations based on family history of CVD or hypercholesterolemia will fail to detect a substantial number (17% to 90%) of children who have elevated lipid levels.
Universal screening will undoubtedly detect those with undiagnosed heterozygous FH or more marked FCHL, who will require more intensive treatment, usually drug therapy. In a recent meta-analysis of screening for FH in a primary care setting, use of TC detected 88%, 94%, and 96% of cases, with false-positive rates of 0.1%, 0.5%, and 1%, respectively. This approach might usefully be combined with a case-finding strategy in relatives of patients with FH.
Identification of children and adolescents affected with hypercholesterolemia through universal screening may bring to attention their adult relatives who will have greater coronary mortality than relatives of children with normal cholesterol levels. If universal lipid screening is combined with an assessment of obesity and high blood pressure, this can also lead to the detection of additional relatives from families at high risk for CVD.
CVD risk factors cluster in childhood and persist into adulthood. Whereas offspring of parents with CVD generally have higher LDL-C and TG and lower HDL-C in both childhood and young adulthood, the majority of children with dyslipidemia and multiple risk factors will be missed by selective screening.
There are some practical problems with universal screening (see later). As well, no longitudinal studies to date are available (and are unlikely ever to be available) to document that starting lipid treatment in childhood decreases adult CVD. One might argue therefore that universal screening seems all the more urgent, given the epidemic of obesity and the metabolic syndrome in our youth.
What are potential concerns about universal lipid screening in childhood? The use of TC in childhood to predict TC or LDL-C in young adults, sufficiently high to warrant treatment, is often associated with suboptimal sensitivity, specificity, and predictive power of a positive test result. For example, if one uses the 75th percentile (about 170 mg/dL) as a lower TC cut point (see Table 12-3 ), the sensitivity (proportion of affected subjects identified) is higher and the specificity (proportion of normal subjects identified as normal) is lower, as is the predictive value of a positive test result. If one increases the cut point for TC to the 95th percentile (about 200 mg/dL), the sensitivity decreases (more children are missed who are destined to be “affected” as adults), but the predictive power of a positive test result increases (more test results above 200 mg/dL correspond to adults who will require treatment).
When one uses quantitative traits such as LDL-C values for screening, there is no simple resolution of this problem. The use of high LDL-C (≥130 mg/dL) rather than high TC as a cut point improves the sensitivity in those with low HDL-C and the predictive power of a positive test result in those with high HDL-C.
A number of longitudinal studies found that when the 75th percentile for TC in children is used as a screening cut point, about half the individuals who will require treatment as adults are identified by universal lipid screening. In one report, the sensitivity was much lower when screening was performed during adolescence, presumably reflecting the temporary shift of LDL-C to lower values during this period of rapid growth and development.
Another unanswered question is whether the detection of elevated TC or LDL-C in children and young adults predict those who are destined to manifest premature CVD. In the Princeton Lipid Research Clinics Prevalence follow-up study of about 30 years, the number (n = 20) of CVD events was small; the sensitivity of childhood LDL-C for prediction of adult CVD was 10.5% and specificity was 81%. Use of family history information does not substantively improve these results. A combined approach using other CVD risk factors, such as obesity, as part of the screening paradigm for future CVD will probably improve the detection of those adults more likely to develop premature CVD.
Health care providers need to be aware of the negative impact of labels on a child. Problems can be created where no problems may have been. Labeling can have a negative impact on a child’s self-esteem, potentially predisposing one to a life that may have been different had the labeling never occurred.
Universal screening raises additional logistic issues. How will pediatricians and family practitioners handle the detection of many children and adolescents with dyslipidemias? Who will counsel in regard to dietary changes, weight loss, and regular exercise habits? Will universal screening, treatment of affected children, and follow-up be cost-effective? Clearly, national resources will be required to change the way pediatric medicine is practiced.
What to Measure
A child does not have to be fasting for TC or HDL-C to be measured. For selective screening, a lipoprotein profile after an overnight fast of 10 to 12 hours is measured for screening youth with a positive family history of premature CVD or dyslipidemia or who have obesity, have multiple CVD risk factors, or are suspected of having secondary dyslipidemia. Such a profile includes TC, TG, LDL-C, HDL-C, and non–HDL-C.
Levels of lipoproteins are typically measured and expressed in terms of their cholesterol content. LDL-C is calculated from the Friedewald equation: LDL-C = TC − (HDL-C + TG/5). Total TG in the fasting state divided by 5 is used to estimate the levels of VLDL-C. If the TG is ≥400 mg/dL, this formula should not be used and a direct LDL-C or apo B should be measured. If the patient is nonfasting, TC, HDL-C, and non–HDL-C levels can be measured. Non-HDL and apo B are valid in the nonfasting state.
Apo B and apo A-I can also be determined by well-standardized immunochemical methods. Such measurements might provide additional useful information, particularly in youth with premature CAD in their parents. Age- and gender-specific cut points for apo B and apo A-I empirically derived from the NHANES sample are available, providing cut points that can be used to define elevated apo B and low apo A-I (see Table 12-3 ). Apo B provides an assessment of the total number of apo B–containing lipoprotein particles.
Non–HDL-C is determined by subtracting HDL-C from TC. Non–HDL-C reflects the amount of cholesterol carried by the “atherogenic” apo B–containing lipoproteins—VLDL, IDL, LDL, and Lp(a)—and is strongly correlated with apo B. In adults, non–HDL-C is a better independent predictor of CVD than LDL-C is. In children, non–HDL-C is at least as good a predictor as LDL-C of the expression of dyslipidemia in adulthood. Percentiles for non–HDL-C in children are available from Bogalusa (see Table 12-3 ).
Advanced lipoprotein testing to determine plasma levels of VLDL, LDL, and HDL subclasses has been performed in children and adolescents by nuclear magnetic resonance spectroscopy or by vertical-spin density-gradient ultracentrifugation in research studies (see also later). However, cut points derived from these methods for the diagnosis and treatment of dyslipidemia in youth are currently not available.
For universal screening, the simplest approach appears to be the measurement of TC, HDL-C, and non–HDL-C in nonfasting specimens. However, treatment algorithms in pediatrics are usually focused on fasting LDL-C. TG is usually assessed as part of the dyslipidemic triad and is often elevated in obesity and the metabolic syndrome. Thus, in an ideal screening program, follow-up TC, TG, LDL-C, HDL-C, and non–HDL-C in the fasted state would be assessed after the initial nonfasting screen.
When to Sample for Dyslipidemia
Cholesterol levels are reasonably consistent after 2 years of age. Cholesterol levels are not routinely measured before the age of 2 years because no formal treatment is recommended for this age group. Ten years of age (range, 9 to 11 years) has been proposed as a good time to obtain a standard lipoprotein profile. Children are older and are able to fast easier, the values are predictive of future adult lipoprotein profiles, and puberty has usually not yet started.
Because TC and LDL-C fall 10% to 20% or more during adolescence, children at risk for familial dyslipidemias should ideally be screened before adolescence, between 2 and 10 years of age. In FH heterozygotes, there is a significant fall in the 1:1 ratio of affected to normal during adolescence. If sampling occurs during adolescence and results are abnormal, levels are likely to be even higher after teenage years. If results during puberty are normal, sampling will need to be repeated toward the end of adolescence (16 years of age for girls and 18 years of age for boys).
The complete phenotypic expression of some disorders, such as FCHL, can be delayed until adulthood, and therefore continued evaluation of subjects from high-risk families with FCHL should occur well into adulthood. Elevated apo B is the first expression of FCHL in adolescents and young adults.
Primary Versus Secondary Dyslipoproteinemia
Before a dyslipoproteinemia is considered to be primary, secondary causes must be excluded ( Table 12-4 ). Each child with dyslipidemia should undergo routine blood testing to rule out secondary causes, including a fasting blood glucose concentration and kidney, liver, and thyroid function tests. In secondary dyslipidemia, the associated disorder producing the dyslipidemia should be treated first in an attempt to normalize lipoprotein levels. If an abnormal lipid level persists, for example, as it often does with diabetes mellitus type 1 and nephrotic syndrome, the patient requires dietary treatment and, if indicated, pharmacotherapy with use of the same guidelines as in primary dyslipidemias.
Lifestyle | Medication |
Obesity | Oral contraceptives |
Physical inactivity | Glucocorticoids |
Diet rich in fat and saturated fat | Anabolic steroids |
Alcohol intake | 13- cis -Retinoic acid |
Endocrine and metabolic | Thiazide diuretics |
Diabetes mellitus | Anticonvulsants |
Metabolic syndrome | Antipsychotics |
Hypopituitarism | Estrogen |
Hypothyroidism | Testosterone |
Pregnancy Polycystic ovarian syndrome | Immunosuppressive agents (cyclosporine) |
Lipodystrophies | Protease inhibitors |
Glycogen storage disease | Others |
Acute intermittent porphyria | Anorexia nervosa |
Renal | Cancer survivor |
Chronic renal failure | Burns |
Hemolytic-uremic syndrome | Idiopathic hypercalcemia |
Nephrotic syndrome | Kawasaki disease |
Hepatic Biliary atresia Alagille syndrome Cirrhosis Hepatitis | Klinefelter syndrome |
Progeria (Hutchinson-Gilford syndrome) | |
Rheumatoid arthritis | |
Systemic lupus erythematosus | |
Werner syndrome |
Guidelines for Treatment of Dyslipidemia in Children and Adolescents
General guidelines for dietary and pharmacologic treatment of primary and secondary dyslipidemias in youth are presented first. Specific therapies relevant to each inherited disorder of dyslipidemia are addressed in subsequent sections of this chapter.
Dietary Therapy
The first approach to therapy for children with dyslipidemia is a modified diet containing decreased amounts of total fat, saturated fat, trans -fat, and cholesterol. The intake of complex carbohydrates is increased, and that of simple sugars is decreased. No decrease in total protein is recommended. Adequate calories should be provided to maintain normal growth and development.
The NCEP Pediatric Panel recommended diet treatment after 2 years of age. Recent data from randomized clinical trials in general populations, such as STRIP, indicate that a diet low in total fat, saturated fat, and cholesterol may be instituted safely and effectively at 6 months of age (see also later).
When to Initiate Dietary Treatment
If the first fasting lipoprotein profile indicates that TC, LDL-C, non–HDL-C, or TG is elevated or the HDL-C is low (see Table 12-3 ), a repeated profile is obtained at least 3 weeks apart to confirm the first profile. If the dyslipidemia persists (i.e., one or more of the lipid or lipoprotein values remains elevated or the HDL-C is low), secondary causes of dyslipidemia (see Table 12-4 ) are ruled out and dietary treatment is initiated. A Step I diet is usually started, and the lipoprotein profile is rechecked in 6 to 8 weeks. If the dyslipidemia persists, a Step II diet is begun.
Both diets require the optimal input of a registered dietitian. This may not always be available, and the health care provider, such as the physician or nurse, may need to provide the basis for the diets in Table 12-5 along with printed materials that are available from the American Heart Association. As well, the daily estimated calories and recommended servings for grains, fruits, vegetables, and milk or dairy according to age and gender are available in tabular form from the most recent publication of the American Academy of Pediatrics. Assessment of dietary intakes may become possible in the future by use of the web-based program developed by the National Cancer Institute.
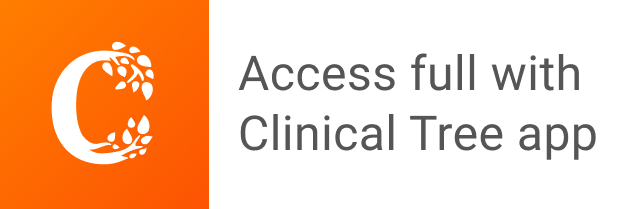