Diseases of the Pericardium, Restrictive Cardiomyopathy, and Diastolic Dysfunction
Allan L. Klein
Craig R. Asher
Overview
Diseases of the pericardium and restrictive cardiomyopathies cause impairment of the diastolic function of the heart. Myriad underlying etiologies, via several mechanisms, result in final common clinical presentations, making specific diagnosis difficult. Historically, these disorders have been within the realm of invasive cardiac investigation (1); however, they have recently become illuminated by several evolving noninvasive techniques, including echocardiography (2,3) and magnetic resonance imaging (MRI) (4). This chapter discusses the general principles of diastolic heart failure including its biology and physiology, clinical presentation, epidemiology, investigation, and treatment. It also reviews specific diseases of the myocardium and pericardium that may cause diastolic dysfunction.
General Principles of Diastolic Heart Disease
Definition of Diastolic Dysfunction
Congestive heart failure is most often secondary to impairment of left ventricular systolic function. Diastolic heart failure is increasingly common and can be associated with significant morbidity and mortality (5). Clinically, it is important to distinguish these conditions, although in a given patient, combined systolic and diastolic dysfunction is frequently observed (6,7). Table 27.2 compares the structure and function of systolic and diastolic heart failure. The main differences are that in systolic heart failure, there is LV cavity enlargement, eccentric hypertrophy and abnormal systolic and diastolic function. While in diastolic heart failure, there is normal cavity sizes, concentric hypertrophy and abnormal diastolic function (7a). Several pathophysiologic definitions of diastolic heart failure have been proposed: (a) impaired capacity of the ventricles to fill without a compensatory increase in left atrial pressure (8); (b) abnormal ventricular filling that would produce inadequate cardiac output with a mean pulmonary venous pressure of less than 12 mm Hg (9), and (c) resistance to filling of one or both ventricles with an inappropriate upward shift of the pressure–volume loop, especially with exercise (10). These definitions have in common an abnormal resistance to filling, causing elevated left-sided filling pressures and congestion. Diastolic dysfunction impairs filling of the ventricle by impairing relaxation (early diastole), reducing compliance (early to late diastole), or leading to external constraint from the pericardium (10,11,12). Many pathologic processes and disease states can produce the clinical constellation of diastolic dysfunction (Table 27.1).
Diagnostic criteria for patients with diastolic heart failure have been proposed as a framework for designing and implementing treatment trials. To diagnose diastolic heart failure, the 1998 European Study Group on Diastolic Heart Failure required (a) clinical evidence of congestive heart failure, (b) normal or mild left ventricular dysfunction, and (c) the presence of impaired relaxation, filling, or compliance (13) (Table 27.2). All three components, as assessed by noninvasive testing or cardiac catheterization, were necessary for a diagnosis. Vasan
and Levy subsequently proposed less rigid criteria using similar inclusions but defining diastolic heart failure as definite, probable, or possible depending on the number of elements present (14). There is a controversy whether measurements of LV relaxation or compliance by cardiac catheterization or Doppler echocardiography are actually needed in the presence of LV hypertrophy or concentric remodeling (14a).
and Levy subsequently proposed less rigid criteria using similar inclusions but defining diastolic heart failure as definite, probable, or possible depending on the number of elements present (14). There is a controversy whether measurements of LV relaxation or compliance by cardiac catheterization or Doppler echocardiography are actually needed in the presence of LV hypertrophy or concentric remodeling (14a).
TABLE 27.1 Conditions involving diastolic heart failure | |||||||||||||||||||||||||||||
---|---|---|---|---|---|---|---|---|---|---|---|---|---|---|---|---|---|---|---|---|---|---|---|---|---|---|---|---|---|
|
Pathophysiology of Diastolic Function
Phases of Diastole
Diastole is the period from the closure of the aortic valve to the end of mitral inflow; it is divided into two periods, an isovolumic relaxation period and an auxotonic period, which includes rapid filling, diastasis (slow filling), and atrial systole (15). These phases are shown in Figure 27.1 relating left ventricular and left atrial pressure tracings and mitral, tricuspid, pulmonary, and hepatic venous Doppler echocardiography profiles. Each phase may be influenced by multiple factors including the effects of myocardial relaxation (16), the passive filling characteristics of the left ventricle (17), the dynamics of the left atrium (18,19,20), characteristics of the pulmonary veins (21) and the mitral valve (22), and the heart rate (23).
Isovolumic Relaxation
The isovolumic relaxation time period occurs from the time of aortic valve closure to mitral valve opening with no change in left ventricular volume (24). During this period, active, energy-dependent myocyte relaxation occurs until mid-diastole. Ventricular pressure (Pv) decay during the isovolumic period follows an approximately exponential curve that can be characterized by its starting pressure (P0) and its time constant (tau, τ) (25). This curve can be fitted with the assumption of either a zero asymptote, Pv = P0e-t/τ (25), or a nonzero asymptote, Pv = P0e-t/τ + Pb (to account for subatmospheric pressure and the effects of pericardial pressure) (9,26), where Pb is the left ventricular pressure at the time of aortic closure or peak negative dp/dt, t is the time after the onset of relaxation, and τ is the time constant of relaxation.
A low value of τ is indicative of fast relaxation, whereas a high value represents slow relaxation. The duration of isovolumic duration can be obtained invasively and by several noninvasive modalities including phonocardiography and M-mode and Doppler echocardiography (27,28,29). Newer measures, including the color M-mode slope (Vp) (30) and the annular early velocity of tissue Doppler echocardiography, have been shown to relate closely to the time constant of relaxation (31). Isovolumic relaxation ends when the left ventricular pressure falls below the left atrial pressure, resulting in mitral valve opening and the start of the rapid-filling phase (9).
Rapid Filling
The auxotonic period occurs from mitral valve opening until mitral valve closing (15). The rapid-filling phase begins at mitral valve opening when the left ventricular pressure falls below the left atrial pressure. Left ventricular pressure continues to fall because of ongoing relaxation and elastic recoil, with blood accelerating due to the development of a left-atrial-to-left-ventricular pressure gradient (9). This gradient is influenced by the level of left atrial pressure at mitral valve opening and the rate of decline of left ventricular pressure (32). Blood rapidly enters the left ventricle from the left atrium during the early filling period, with approximately 70% of the stroke volume received by the left ventricle during the first one third of diastole (2). Active ventricular suction (pulling of blood) may play an important role in the rapid-filling phase (33,34). Ventricular suction may occur because of the recoil of the compressed elastic elements during contraction (35). Rapid filling ends as atrial and ventricular pressures equilibrate (9).
Diastasis (Slow Filling)
Diastasis, or the slow-filling period, occurs between the rapid-filling phase and atrial contraction and accounts for less than 5% of filling. After rapid filling, left atrial and ventricular pressures are almost equal, yielding no immediate forward driving gradient (9).
Atrial Filling (Contraction)
The atrial filling phase of diastole occurs when the left atrial pressure rises above the
ventricular pressure, forcing or pushing blood across the mitral valve and a small amount of regurgitation into the pulmonary veins. This late diastolic transmitral flow increases ventricular end-diastolic volume by 25% in normal individuals with only a small rise in mean pulmonary venous pressure. Diastole ends with onset of ventricular systole when the rapid increase in ventricular pressure closes the mitral valve (9).
ventricular pressure, forcing or pushing blood across the mitral valve and a small amount of regurgitation into the pulmonary veins. This late diastolic transmitral flow increases ventricular end-diastolic volume by 25% in normal individuals with only a small rise in mean pulmonary venous pressure. Diastole ends with onset of ventricular systole when the rapid increase in ventricular pressure closes the mitral valve (9).
TABLE 27.2 Comparison Between SHF and DHF: LV Structural and Functional Features | ||||||||||||||||||||||||||||||||||||||||||||||||||||||||||||||||||||||||||||||||||||||||||
---|---|---|---|---|---|---|---|---|---|---|---|---|---|---|---|---|---|---|---|---|---|---|---|---|---|---|---|---|---|---|---|---|---|---|---|---|---|---|---|---|---|---|---|---|---|---|---|---|---|---|---|---|---|---|---|---|---|---|---|---|---|---|---|---|---|---|---|---|---|---|---|---|---|---|---|---|---|---|---|---|---|---|---|---|---|---|---|---|---|---|
|
Determinants of Diastolic Function
Diastolic function depends on four major factors: (a) active myocardial relaxation, (b) passive pressure–volume relationships (i.e., left ventricular compliance), (c) left atrium (including atrial function), pulmonary vein, and mitral valve characteristics, and (d) heart rate (9). Each variable can in turn be affected by different physiologic states and organic heart diseases. The physiology of ventricular diastolic function is exceedingly complex, involving interactions at the subcellular, myocyte, myocardial tissue, and whole-chamber levels (11,36). Pericardial contributions to diastolic physiology are discussed later in the chapter.
Myocardial relaxation is mediated by intracellular ATP and calcium (37,38). Contraction is initiated by calcium release in large quantities from the sarcoplasmic reticulum. This free ionized calcium ion binds to the troponin–tropomyosin complex, removing the inhibitory effect of that complex on the actin–myosin myofilaments (39). Active cross-bridges form between actin and myosin with consequent contraction of the myofilaments. This rapid increase of intracellular calcium is countered
by calcium sequestration into the sarcoplasmic reticulum by the calcium-ATPase pump (40), which is tonically inhibited by the phospholamban. It has been demonstrated that relaxation is asynchronous, occurring earliest in apical segments and resulting in the intracavitary suction of blood from mid and basal regions toward the apex (41). Because relaxation is an energy-dependent process, it is influenced by many factors, including ischemia and left ventricular mass (42,43,44).
by calcium sequestration into the sarcoplasmic reticulum by the calcium-ATPase pump (40), which is tonically inhibited by the phospholamban. It has been demonstrated that relaxation is asynchronous, occurring earliest in apical segments and resulting in the intracavitary suction of blood from mid and basal regions toward the apex (41). Because relaxation is an energy-dependent process, it is influenced by many factors, including ischemia and left ventricular mass (42,43,44).
The passive filling characteristics of the ventricle describe the late diastolic volume–pressure relationship of the chamber. This is determined by the viscoelastic nature of the myocardium, the chamber size, the shape and thickness of the wall, the right and left ventricular pressure–volume interaction, the intrathoracic pressure and pericardial restraint, and any active myocardial relaxation that remains incomplete (17). Compliance, the ratio of change in volume per change in pressure, quantifies the net result of all of these factors. Intrinsic myocardial stiffness relates to the amount of collagen within the myocardium. Collagen plays an essential role in converting the contractile force of the myocytes into intraventricular pressure, as well as determining overall ventricular size and shape (45,46). Collagen fibrils coil around myofibrils. Little force is needed initially to stretch the coil, whereas large forces are required at full stretch, yielding an exponential stress–strain relationship (47). Pressure-induced hypertrophy is associated with increased collagen content and secondarily increased overall stiffness. Local collagen scarring after myocardial infarction is threefold stiffer than the surrounding myocardium (48).
Diastolic properties of the ventricle have been studied and quantified extensively using pressure–volume loops (Fig. 27.2) (49,50,51). Simultaneous recording of ventricular pressures and volumes (contrast angiography, radionuclide imaging, or echocardiography) yields the loops, which have traditionally been the benchmarks for quantifying the systolic and diastolic function of the heart.
There is a curvilinear relationship between the volume and pressure in the ventricle that is determined by the stress–strain relationship. As the volume increases in the left ventricle during diastole, there is an increase in left ventricular (LV) pressure (47). The slope of the pressure–volume curve during diastole (dp/dV) is the chamber stiffness, and the inverse of this relation is the chamber compliance (52). The slope (dp/dV), or stiffness, of the left ventricle is not constant but rather follows an exponential curve upward as volume increases. Thus, when end-diastolic volume increases without intrinsic change in the mural stress–strain relationship, stiffness increases along this exponential curve. Conversely, an increase in stiffness constant E will move the loop to a steeper curve with no change in volume. Finally, it can be seen that increasing the loading pressure without changing the volume moves the loop directly upward with no change in dp/dV or stiffness.
Pulmonary vein/left atrium and mitral valve characteristics determine the volume of blood that enters the left ventricle (9). Early rapid filling is largely dependent on the pressure gradient between the pulmonary veins/left atrium and the left ventricle and the rate of myocardial relaxation and ventricular suction (33,34,53,54). Diseases of the mitral valve, such as mitral stenosis, delay left ventricular filling and prevent early rapid filling (9).
The left atrium acts as a reservoir of blood, as a passive conduit during early LV filling, and as an active pump at end-diastole (55). Atrial systolic function (active phase) may be essential to maintaining cardiac output in disease states (56,57). Atrial function relates to its compliance (58), preload, afterload, and intrinsic contractility. Atrial contractile performance varies considerably in health and disease (56,57). In young and healthy normal individuals, the atrial contribution is less than 20% of the total volume, whereas in older normal individuals, the atrial “kick” accounts for a greater proportion of the total LV filling (59). Atrial contractile function has delayed recovery after electrical cardioversion (20). Decreased atrial systolic function due to infiltration plays a role in the reduction in forward transmitral atrial contraction wave by Doppler echocardiography as seen in advanced amyloidosis (60).
Heart rate bears directly on cardiac output in cases of diastolic dysfunction (23,61). As heart rate increases, the diastolic filling period preferentially decreases with respect to the systolic ejection period. As ventricular filling is functionally delayed, adequacy of inflow deteriorates and cardiac output paradoxically falls.
Neurohormonal activation, conduction abnormalities, and pericardial constraint may also influence diastolic function. Catecholamines may enhance relaxation and increase heart rate, thus decreasing the diastolic period (62). Conduction abnormalities may also influence diastole (63). LV relaxation may be impaired by conduction abnormalities causing segmental asynchrony (64,65). This may contribute to decreased exercise tolerance in patients with left-bundle-branch block or right ventricular paced rhythms. The pericardium (see later discussion) considerably affects diastolic function, notably in constrictive pericarditis (66).
Diastolic Filling Patterns
Extensive literature exists regarding the empirically observed patterns of transmitral flow observed in normal individuals and patients with diastolic dysfunction. These patterns are the end result of the complex events only briefly described earlier and are nonspecific for any particular physiologic perturbation. Diastolic filling patterns may be influenced by technical factors (sample volume placement) and hemodynamic factors (heart rate, volume status, or conduction disorders) and are not always concordant with diastolic function (67).
Most simply, investigators have measured the peak velocity of the E wave and A wave by transmitral pulsed-wave Doppler and their relationship, the E/A ratio (68). These basic
parameters vary with age and within the spectrum of diastolic filling (Fig. 27.3) (59,69). Taken alone, the normal situation (E/A ratio >1) passes through a reversed phase (E/A ratio <1), then through a “pseudonormal” pattern (E/A ratio >1) to the most abnormal “restrictive” pattern (E/A ratio >2). Thus, the E/A ratio demonstrates a U-shaped curve, making it impossible to tell from this single parameter where in the spectrum a given patient lies (31). The pulmonary venous pattern is an important source of added information (21) that may overcome the limitations of the E/A ratio for determining diastolic function in many patients. The finding of an increased pulmonary atrial reversal flow reversal velocity or width as well as a significant change in the E/A ratio with the Valsalva maneuver may aid to differentiate “pseudonormal” from normal diastolic function, corresponding to elevation of mean left atrial or left ventricular diastolic pressures, respectively (69,70,71).
parameters vary with age and within the spectrum of diastolic filling (Fig. 27.3) (59,69). Taken alone, the normal situation (E/A ratio >1) passes through a reversed phase (E/A ratio <1), then through a “pseudonormal” pattern (E/A ratio >1) to the most abnormal “restrictive” pattern (E/A ratio >2). Thus, the E/A ratio demonstrates a U-shaped curve, making it impossible to tell from this single parameter where in the spectrum a given patient lies (31). The pulmonary venous pattern is an important source of added information (21) that may overcome the limitations of the E/A ratio for determining diastolic function in many patients. The finding of an increased pulmonary atrial reversal flow reversal velocity or width as well as a significant change in the E/A ratio with the Valsalva maneuver may aid to differentiate “pseudonormal” from normal diastolic function, corresponding to elevation of mean left atrial or left ventricular diastolic pressures, respectively (69,70,71).
Still, exceptions and limitations to the utility of pulmonary venous flow diminish the capability of distinguishing diastolic flow patterns in all individuals. Some patients may not have adequate pulmonary venous flow profiles; in particular, the atrial reversal wave may not be well delineated. Blunting of pulmonary venous systolic waves may be present in young individuals due to rapid diastolic “suction,” and atrial reversal may be absent in advanced diastolic dysfunction due to the loss of atrial function with amyloid infiltration (69,72). Therefore, other modalities for assessment of diastolic function, including color M-mode Doppler and tissue Doppler echocardiography, have evolved that provide important adjunct information in determining diastolic function and left ventricular filling pressures (31) (Fig. 27.4 and Table 27.3).
Right-Sided Doppler Flows
Diastolic function of the right heart can be evaluated by the interrogation of the tricuspid inflow, hepatic vein flow, and superior vena cava flow (73,74). The Doppler flow patterns in the hepatic veins and superior vena cava are somewhat similar to the pulmonary venous flow patterns (3,75). In addition to the S, D, and AR waves, a second reversed flow is often seen between the S and the D waves at the
end of ventricular contraction (VR) (74). Right-sided flow velocities normally increase during inspiration and decrease with the Valsalva maneuver (74,76).
end of ventricular contraction (VR) (74). Right-sided flow velocities normally increase during inspiration and decrease with the Valsalva maneuver (74,76).
TABLE 27.3 Diastolic filling variables | ||||||||||||||||||||||||||||||
---|---|---|---|---|---|---|---|---|---|---|---|---|---|---|---|---|---|---|---|---|---|---|---|---|---|---|---|---|---|---|
|
As with the pulmonary venous flow, the S wave is related to right atrial (RA) relaxation and tricuspid annular descent and the D is related to early right ventricular (RV) filling velocity (E). The magnitudes of AR and VR vary according to RV stiffness, RA contractility, and central venous pressure (77). The effect of respiration of the right-sided flows is useful in differentiating constriction from restriction and estimating right-sided filling pressures (3,75).
Color M–Mode Doppler
A number of investigators have studied the use of the color Doppler M–mode (CMM) to characterize the spatiotemporal distribution of blood velocity throughout the ventricular inflow region in diastole (30,31,78,79,80,81).
A typical CMM echocardiogram has a temporal resolution of 5 msec, a spatial resolution of 300 μm, and a velocity resolution of approximately 4 cm/second. In normal sinus rhythm from the apical four-chamber view, the CMM demonstrates a characteristic pattern consisting of two filling waves propagating from the left atrium to the left ventricle, corresponding to the E and A waves. The velocities are highest above the mitral valve and lowest as they approach the apex, as shown by the change in color. The onset of flow occurs earlier at the mitral valve level than at the apex (31). The flow does not propagate instantaneously but rather propagates with a velocity (Vp) given by the slope of the leading edge of the blood wave.
Several qualitative and quantitative parameters have been proposed to characterize transmitral filling by the CMM echocardiogram. These studies have been used to characterize ventricular relaxation compared to cardiac catheterization (30,82). Brun et al. identified the leading edge of the E wave as representing the propagation velocity (30). This parameter is measured by identifying the boundary of the leading edge of color as it propagates into the ventricle, drawing a tangent to this line, and measuring its slope on the M-mode. A negative correlation was demonstrated between this propagation velocity and the time constant of relaxation τ in patients with coronary disease and cardiomyopathy. This propagation velocity parameter has been reported to be altered in conditions of delayed relaxation and ischemia, with a more rapid fall in ventricular pressure allowing a greater propagation of flow into the left ventricle (30,82). Another approach quantifying the propagation velocity (78) measured the delay required for the point of maximum velocity to traverse from the mitral valve to the apex.
In contrast to the standard mitral inflow velocities, the CMM propagation velocity has been determined to be relatively preload independent, as validated by hemodynamic measurements in humans and animals (80,83). When coupled with the ratio of the mitral inflow E wave to the CMM propagation velocity in intensive care unit patients, it provided a good estimate of pulmonary capillary wedge pressure (PCWP) (84). A study showed that an E/Vp ratio of 1.5 or greater was the best predictor of in-hospital clinical heart failure in patients with their first myocardial infarction (81). It appears that the propagation velocity may also be a discriminating feature between restrictive and constrictive diseases. Constrictive pericarditis is characterized by relatively normal early diastolic relaxation (and thus early diastolic filling) with an abrupt mid-diastolic cessation of inflow. Restrictive diseases have abnormal relaxation and impaired inflow from the opening of the mitral valve. These pathophysiologies seem to be reflected in the propagation velocity profile. Constrictive pericarditis is associated with extremely rapid flow propagation (a slope of >100 cm/sec), whereas restrictive cardiomyopathy shows markedly slowed propagation of the E wave (75,85).
Tissue Doppler imaging
Tissue Doppler imaging (TDI) quantifies the low velocity and high amplitude of tissue such as the ventricular myocardium or the motion of the mitral annulus (86,87,88). It provides a detailed spatial map of velocity in the myocardium, which can be further temporally enhanced with the CMM Doppler and pulsed-wave Doppler.
The TDI myocardial velocities are composed of several waves. After the electrocardiographic QRS, a short-amplitude multiphasic signal coincides with the closure of the mitral and tricuspid valve (89) and is caused by translational motion and geometric LV changes, assuming a more spherical shape during isovolumic contraction. After this signal, a larger, positive wave represents ventricular systole (SM). A second, multiphasic signal during isovolumic relaxation coincides with aortic valve closing and tricuspid valve opening. In sinus rhythm, two waves corresponding to early filling (EM) and atrial contraction (AM) appear as a mirror image of the mitral inflow early (E) and atrial (A) filling velocities. In normal subjects, the peak of the EM occurs earlier than the peak mitral inflow E wave (20 msec), suggesting that fast relaxation of the myocardium generates suction that pulls blood into the ventricle (E wave).
However, in patients with diastolic dysfunction, the mitral inflow E wave coincides with or precedes the EM, suggesting more passive filling (31).
However, in patients with diastolic dysfunction, the mitral inflow E wave coincides with or precedes the EM, suggesting more passive filling (31).
A major limitation of TDI is the effect of translation and rotation of the heart, in particular from the parasternal long-axis view. Thus, motion of the LV myocardium and annulus in the longitudinal plane can be measured from the apical four-chamber view. Because the apex is relatively fixed throughout the cardiac cycle and the axial plane of motion of the LV myocardium is parallel with the axis of the transducer, the velocities obtained from this acoustic window represent motion secondary to contraction and relaxation and do not require angle correction (31).
Color Doppler TDI provides a color map with high spatial resolution that is able to provide velocity data simultaneously from multiple segments of the myocardium. In contrast, pulsed-wave TDI is much simpler, providing high temporal and velocity resolution, with the data being stored using digital or video format. A limitation is that it measures only one point in time compared to the multiple information obtained from color Doppler TDI (90).
In normal individuals, the TDI velocities are mirror images of the mitral inflow velocities. With the aging process, there is an inverse effect on the EM/AM ratio similar to that reported for the mitral inflow (89). However, there are significant differences in patients with organic disease, distinct from transmitral flow velocities (91). In patients with impaired relaxation, the EM velocity decreases when the EM/AM ratio is less than 1. In patients with advanced diastolic dysfunction (see later discussion) the EM remains low even in the presence of elevated filling pressures (89).
Tissue Doppler EM is less affected by preload than standard Doppler LV filling indices similar to CMM, and it has been shown to correlate with the time constant of relaxation in patients with normal or increased preload (92,93,94). In hemodynamic studies performed by Oki et al. the time constant of relaxation correlated well with EM in all patients independent of filling pressures (83,92,93,95). In patients with various degrees of diastolic function, the tissue Doppler EM was the best discriminator between normal individuals (16 cm/second) and patients with abnormal diastolic function (7.5 cm/second) compared to the standard mitral inflow or pulmonary vein flow (96). Other groups have confirmed that a TDI EM of 8 cm/second is a useful cut-off between normal and abnormal filling pressures (97).
TDI myocardial velocities can be used to differentiate constrictive pericarditis from restriction. In patients with restriction, both relaxation and compliance is abnormal, whereas relaxation is normal in constriction. Patients with restriction have very slow early diastolic motion of the annulus, whereas patients with constriction have normal or very rapid early diastolic annular velocity (>8 cm/sec), suggesting preserved relaxation (85,86).
Strain imaging is another new modality that measures the deformation properties of a myocardial segment independent of translation or tethering. It is given by the equation S = (L0 – L1)/L0, where L0 is initial length and L1 is compressed length (87,98). The strain rate measures the velocity in a certain direction over time dv/dx (sec-1) of the longitudinal or circumferential fibers of the myocardium from parasternal or apical views. This technique has been used in patients with various diseases including hypertrophic cardiomyopathy (99) and patients with coronary artery disease (87,100,101,101a).
Interpretation of Diastolic Filling Patterns
Based on information from mitral inflow, pulmonary vein flow, CMM, and TDI, four patterns of diastolic function can be determined (Fig. 27.4). These patterns depend on age and hemodynamic conditions and represent stages of relaxation and compliance abnormalities and filling pressures. A recent classification proposed that diastolic dysfunction be graded in four stages that correlate with diastolic impairment and symptom class (102) (Fig. 27.5).
Normal
The normal filling pattern is seen in normal individuals with normal relaxation, compliance, and filling pressures. Normal individuals demonstrate a briskly accelerating E
wave, relatively rapid deceleration, and an A wave significantly smaller in magnitude than the E wave. The E/A ratio is greater than 1, the mitral deceleration time is typically between 150 and 220 msec, and the isovolumic relaxation time (IVRT) is greater than 100 msec. The S/D ratio is generally greater than 1, and the AR wave should be less than 35 cm/second. The CMM propagation is relatively fast (>45 cm/sec), and the tissue Doppler EM is greater than 8 cm/sec, consistent with normal ventricular relaxation (31).
wave, relatively rapid deceleration, and an A wave significantly smaller in magnitude than the E wave. The E/A ratio is greater than 1, the mitral deceleration time is typically between 150 and 220 msec, and the isovolumic relaxation time (IVRT) is greater than 100 msec. The S/D ratio is generally greater than 1, and the AR wave should be less than 35 cm/second. The CMM propagation is relatively fast (>45 cm/sec), and the tissue Doppler EM is greater than 8 cm/sec, consistent with normal ventricular relaxation (31).
In young normal individuals, there is a greater predominance of early diastolic filling due to the rapid “suction” effect of the ventricle (more enhanced relaxation with Vp >55 cm/second and EM >10 cm/second) and little additional filling during atrial contraction. This results in an even greater E/A ratio with further shortening of the deceleration time. Because atrial contribution is minimal, the mitral inflow A wave and pulmonary vein AR are very small. The S/D ratio may be less than 1, representing the large contribution of early filling.
Delayed relaxation pattern (stage I)
This pattern is seen in patients with delayed LV relaxation but with relatively normal compliance and filling pressures. As a consequence of normal aging and various pathologic states with early stages of diastolic dysfunction, the time constant of relaxation is lengthened, and the E wave shows a slower acceleration, a lower peak velocity, and a prolonged deceleration time (>220 msec) and isovolumic relaxation time (>100 msec). To the extent that emptying may not be complete by the end of diastasis, an increased left atrial volume will be present at the time of atrial contraction, leading to a larger A wave, compensating in large part for the smaller E wave. Thus, the E/A ratio is less than 1, and concomitantly the S/D ratio is greater than 1 because if E is small, the corresponding D is small. Because relaxation is impaired, the color M-mode Vp is prolonged (<45 cm/second) and EM is decreased (<8 cm/second).
Impaired relaxation may occur with normal or elevated filling pressures. If left ventricular end-diastolic pressure is normal, pulmonary vein AR is less than 35 cm/second, though if it is elevated, the AR is greater than 35 cm/second, or the duration of AR is greater than the duration of the mitral inflow A (103,104).
This pattern is seen in normal aging (3,59), ischemia (105), and hypertrophic cardiomyopathy (106,107) and secondary hypertrophy (108), and is usually seen in patients who typically have only mild symptoms or are asymptomatic (71). It can also be seen with hypovolemia due to a decreased left-atrial-to-left-ventricular pressure gradient with greater filling during atrial systole (75,109).
“Pseudonormal” pattern (stage II)
This pattern is difficult to recognize because it is similar to the “normal” pattern. As left atrial pressure increases further to compensate for deteriorating diastolic function, the peak E-wave velocity increases. Therefore, abnormalities of relaxation and compliance and elevated filling pressures are present. This is associated with a normal appearance of the transmitral inflow with an E/A ratio between 1 and 2, a deceleration time between 150 and 220 msec, and an isovolumic relaxation time between 60 and 100 msec (69,71,75). One important feature to distinguish this pattern from the normal one is the pulmonary venous AR wave, which in pseudonormal filling displays a prolonged and increased AR of greater than 35 cm/second (59), whereas the pulmonary vein S/D ratio could be normal or less than 1. The AR wave prominence may not be present if atrial systolic failure occurs, although the duration of AR may still be greater than that of the mitral A wave. In addition, the Valsalva maneuver may be useful in differentiating normal from “pseudonormal” by reducing the preload, causing the normal-appearing E/A ratio to be less than 1, and thus exposing a delayed relaxation pattern (3,69,70,110). CMM propagation velocities and TDI are particularly helpful in detecting pseudonormal filling with a Vp of less than 45 cm/second and an EMof less than 8 cm/second (96).
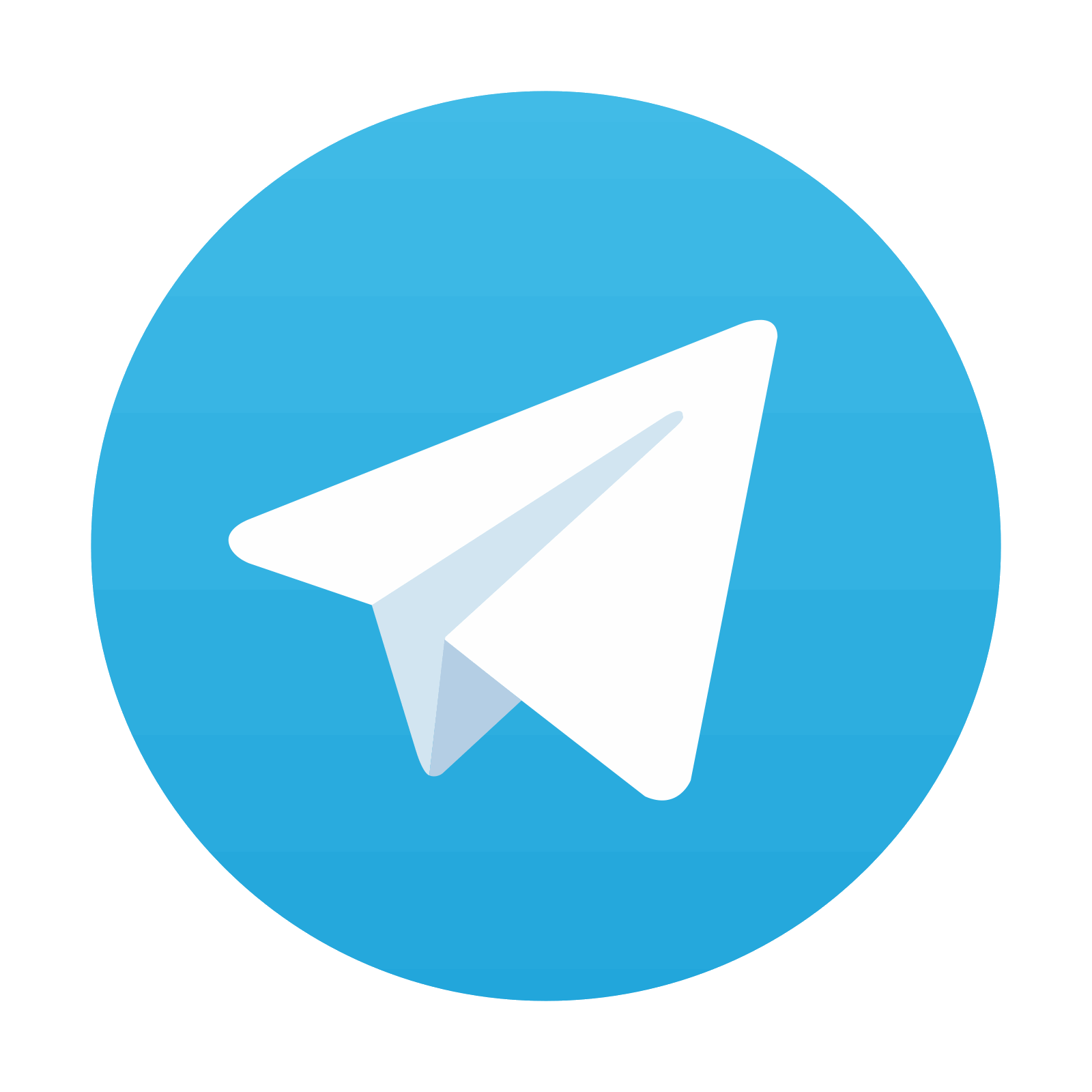
Stay updated, free articles. Join our Telegram channel
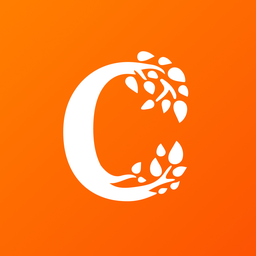
Full access? Get Clinical Tree
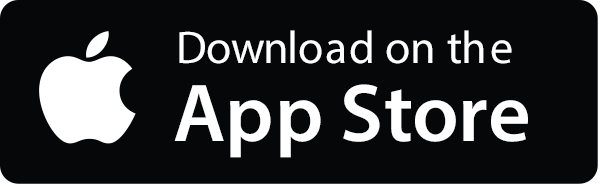
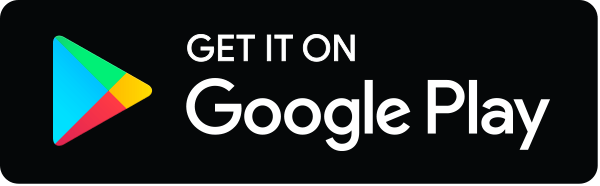
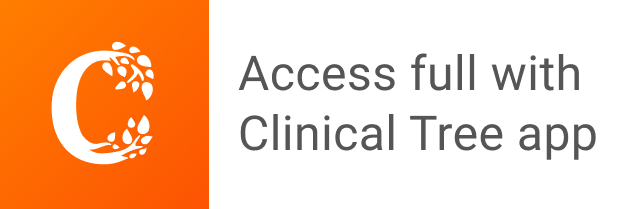