Tracheoesophageal fistula/esophageal atresia
Congenital acinar hypoplasia
Congenital alveolar hypoplasia
Surfactant deficiency
Bronchopulmonary dysplasia
Cystic fibrosis
Neuroendocrine hyperplasia of infancy
Chronic obstructive pulmonary disease
Asthma
Idiopathic pulmonary fibrosis
Infectious disease
Cystic fibrosis (CF), the most common lethal genetic disease among Caucasians (1/2000 births), is caused by mutations in the CFTR chloride ion channel resulting in altered ion flux in exocrine organs and hyperproduction of viscous mucus. Impairment of mucociliary function in the conducting airways leads to colonization of pathogenic bacteria and intractable, infectious complications. Path clamp studies on iPS-derived airway epithelial cells have indeed shown altered chloride conductance [25, 26]. These cells could therefore be used for drug screening. More complex three-dimensional (3D) models might begin to explain how altered chloride conductance leads to the observed severe impairment of mucociliary function. Such models do not exist currently, however.
A subset of almost universally lethal pediatric lung diseases are due to surfactant deficiency, caused by mutations in genes encoding surfactant proteins (SP-C, SP-B), or factors required for surfactant trafficking (ABCA3, CSFR2A) [27]. The clinical and pathological features of these diseases vary and are, among others, dependent on the exact mutations. Examining how a mutation affects the function of surfactant producing type II alveolar epithelial (ATII) cells and screening for drugs that might act as potential “correctors” requires modeling using hPSC-derived ATII cells. Several severe congenital pediatric lung diseases are not associated with surfactant deficiency, and their etiology is unknown. Pulmonary acinar dysplasia, for example, is characterized by developmental arrest prior to the canalicular or saccular stage of lung development [28, 29]. In two other diseases, congenital alveolar dysplasia [30] and alveolar growth abnormalities [31], developmental arrest or delay appears to occur later in lung development . Neuroendocrine hyperplasia of infancy is another severe pediatric lung disease of which the etiology and the likely developmental origin are unknown [31, 32]. The availability of iPS-based models of human lung development would shed light on the pathogenesis of pediatric congenital lung disease and might lead to novel therapeutic approaches. Equally importantly, such studies would provide unique insights into normal human lung development and into novel approaches to treat bronchopulmonary dysplasia of prematurely born infants, characterized by decreased alveolarization and impaired secondary septation of alveoli and one of the most threatening problems in prematurely born infants [33].
Directed differentiation of hPSCs into respiratory epithelium would also provide a novel and biologically relevant in vitro model to study infectious disease. Although mouse adapted strains of influenza exist [34], influenza infects humans, ferrets, and birds. Other viruses, such as respiratory syncytial virus, show different cellular tropism in mice and humans [35].
Modeling using hiPSCs might provide important insight into chronic obstructive pulmonary disease (COPD), one of the most prevalent lung diseases. COPD is characterized by, among others, mucous cell metaplasia and hyperplasia. Notch signaling enhances the formation of secretory cell types during development [36], directs differentiation of basal cells, the stem cells of the large airway , towards a secretory fate (club and goblet) [37], and induces mucous metaplasia in human tracheal explants [38]. hiPSC-derived airway cells could be used to examine to what extent this pathway also regulates the overabundance of goblet cells in COPD. Furthermore, hiPS-derived airway cells would allow molecular studies into genetically determined susceptibility to noxious insults [39] and into the role of inflammatory stimuli [39].
Idiopathic pulmonary fibrosis is an intractable, fibrotic lung disease [40, 41] with a survival of 3–4 years. Although recent trials suggest that two drugs (pirfenidone and nintedanib) [42, 43] slow disease progression to some extent, the only definitive treatment is lung transplantation. IPF is characterized by fibroblastic foci and obliteration of alveoli . Genetic predisposition and environmental exposure, such as smoking and metal dust, likely determine disease penetrance [40, 41]. While most cases are sporadic, several genetic polymorphisms and mutations predispose to IPF [44]. The nature of some of these mutations suggests a critical role for ATII cells, the alveolar cells that secrete surfactant . These can replace damaged ATI cells to restore alveolar integrity after injury [45, 46]. Mutation in SP-C and SP-A2 [47, 48], which is selectively expressed in ATII cells, predisposes to IPF, likely by causing aberrant processing of the protein, resulting in an unfolded protein response. It is not known however how the fibrotic process is initiated and sustained. Other mutations or polymorphisms affect genes that are not specifically expressed in ATII cells, such as telomerase [49]. Impaired proliferative potential or function of ATII is therefore likely central to IPF pathogenesis. Another predisposing allele involves the regulatory region of MUC5B, which is not known to be expressed in ATII cells however, and its role in pathogenesis is entirely unknown [50].
ATII cells can currently not be isolated ex vivo and maintained in vitro. ATII cells isolated from patients after diagnosis may not be informative for disease pathogenesis and predisposition, as many observed changes in expression patterns may be secondary to the terminal fibrotic disease process. The mouse furthermore does not model the human disease well. Administration of bleomycin to mice causes patchy fibrosis that resolves over time, and its validity as a model for IPF is debatable [51, 52]. The best opportunity to gain insight into the pathogenesis of IPF is attempting to model this disease using iPSCs.
Finally, one of the most frequent developmental abnormalities of the airway and gastrointestinal tract is tracheoesophageal fistula/esophageal atresia (TEF/EA). TEF/EA can occur in isolation or as part of a variety of syndromes and associations, such as CHARGE, VACTERL, and Opitz syndrome [53]. Although several genetic mouse knockout models show features of TEF/EA, very few of those genes are mutated in humans with this congenital anomaly, and the etiology of most cases of TEF/EA in humans is not known [54].
Lung development and the case for human PSCs: hPSC-derived cells are a discovery tool that complements genetic approaches in the mouse. The availability of adequate numbers of human cells at well-defined stages of development allows genome-wide expression and epigenetic studies as well as RNAi and CRISPR/Cas9 screens for factors involved in lineage specification that are currently not feasible in mouse embryos. A better understanding of human lung development will in turn improve directed differentiation of hPSCs, the use of carefully timed signals that recapitulate development in the mouse [55–62] to induce specification and maturation of hPSCs into a specific lineage [1, 56]. The generation of functional, mature cells is still a major challenge, however. This may in part be due to the fact that in many organs, human and mouse organogenesis diverge more as development proceeds, likely because of a 3000-fold difference in body mass, profoundly affecting metabolism, organ size, architecture, and function and because of their very different habitats and positions in the food web.
The developmental dynamics and architecture of the respiratory system differ in mouse and human [33, 63]. The development of three-dimensional models could shed light on the underlying mechanisms. A pseudostratified epithelium containing basal cells, the stem cells of the airway , extends to terminal bronchioles in the human but is limited to trachea and large airways in mice, where it is replaced more distally by a columnar epithelium. Secretory club cells and chemosensory “tuft” or “brush” cells are more frequent in rodents than in humans, while the opposite is true for mucus-producing goblet cells [13, 64, 65]. Only the proximal airways contain submucosal glands in mice, while these are also found distally in the main bronchi in humans [66]. The architecture of the distal lung , consisting respiratory bronchioles, alveolar ducts, and associated alveoli , and collectively called the acinus, is more complex in humans than in rodents. The timing of milestones in lung development is also different in mice and humans [33, 63]. The saccular stage, where alveolar sacs containing distinguishable ATI and ATII cells form at the end of the stalks generated during the preceding process of branching morphogenesis [64, 67, 68], begins in late gestation, at E17.5, in the mouse, but relatively much earlier, at week 24, in humans [33]. In humans, further exponential postnatal alveolar growth occurs in the first 2 years, and a slower increase in alveolar number continues into puberty. In rodents, virtually all alveolar growth occurs postnatally in the first 20 days of life and stops more abruptly [69].
Finally, modeling human lung development using hPSCs may also shed light on the biology of human adult stem cells . As their identity and the mechanisms underlying their specification are largely unclear, hPSCs may provide a “forward” approach to gain insight into adult stem cells and into mechanisms involved in regeneration .
Lung Development
As recapitulating lung development in vitro is the scientific underpinning of directed differentiation of PSCs, we will first briefly review the critical stages of the development of the respiratory system (Fig. 16.1).
Lung and airway are derived from the definitive endoderm (DE), one of the germ layers that arises during gastrulation (E7 in mouse and in the third week of gestation in humans). Specification into mesoderm vs. endoderm depends on the strength of nodal signaling: high nodal signaling drives a DE fate while cells exposed to lower levels of nodal signaling are fated towards mesoderm [61, 62, 70, 71]. The DE develops into a tube where organ domains are progressively defined through transcriptional and epigenetic mechanisms along the anterior–posterior axis.
Proximodistal specification is indicated by differential expression of the transcription factors Sox2, Hhex, and Foxa2 in the anterior half, while the posterior half is marked by Cdx2 [72]. Retinoic acid, Wnt, and FGF4 are important for posterior endoderm specification [59, 73–75]. Recent studies in hPSCs have shown that blocking TGF-β and BMP signaling promotes an anterior fate [76]. Furthermore, timing of the exit from the primitive streak appears to determine anteroposterior identity [77], suggesting that the initial patterning may at least in part be established during gastrulation.
Organ domain specification is driven by permissive and instructive signals from surrounding tissues [71, 78]. Organ domains may also be prepatterned through epigenetic mechanisms during the early development of DE and of the gut tube, as has been shown for pancreas and liver [79]. The lung field arises on the ventral AFE and is characterized by the expression of Nkx2.1, which is also expressed in the forebrain and the thyroid, which is additionally marked by Pax8 [71]. Canonical WNT, BMP, and FGF10 signaling from the ventral mesoderm to the AFE are involved in the specification of the lung field [80–85] (Fig. 16.2). RA is required for lung bud formation and in the mouse appears to act indirectly through regulation of Wnt, TGF-β, and FGF10 signaling [86–89]. A dorsoventral BMP signaling gradient, established by expression of BMP4 in the ventral mesenchyme and its antagonist, Noggin, dorsally and in the notochord, regulates patterning of trachea and esophagus [64, 90] and establishes a reciprocal dorsoventral Sox2/Nkx2.1 gradient [91] that differentiates the gut endoderm into the dorsal esophagus (marked by Dlx3 and high expression of Sox2) and a ventral trachea (marked by Nkx2.1 and Nkx2.5 and lower expression of Sox2) [64, 92, 93] (Fig. 16.2). Sox2 is subsequently more highly expressed in the proximal airway and plays a critical role in tracheal development and in the maintenance of tracheal and proximal airway epithelium [94]. Foxf1, expressed in mesoderm, required for the correct separation of trachea and esophagus and is induced by endodermally derived Sonic Hedgehog [78, 95].
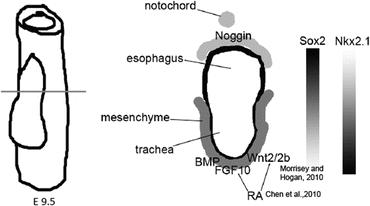
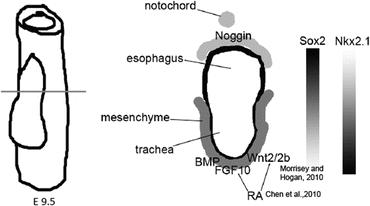
Fig. 16.2
Schematic representation of dorsoventral patterning of the AFE
Subsequently, the lung buds undergo a complex branching process, driven by proliferating progenitors at the tips of the buds [96] (pseudoglandular stage, Fig. 16.1). In the stalks, cell cycle activity ceases, and differentiation of the airway epithelium occurs, with the emergence of basal, goblet, club, ciliated, and other cell types [96] (canalicular stage, Fig. 16.1) SOX2 is expressed in the developing airways, while SOX9 is expressed in the distal tips [97–99]. Cessation of proliferation coincides with loss of SOX9 expression in the mouse [96]. The regulation of branching morphogenesis and subsequent specification and terminal differentiation of specific cell types is extremely complex and involves interactions of multiple signals between the pulmonary endoderm and the surrounding mesenchyme. Among the critical factors secreted by the mesenchyme are FGF10 and BMP4, while Hedgehog signals from the endoderm to the mesenchyme [64]. Canonical and non-canonical Wnt signaling as well as BMP4 are involved in promoting a distal fate [85, 100, 101], while RA and Notch signaling promote a more proximal fate [102, 103]. Notch also enhances differentiation of airway basal cells into secretory and goblet cells, but not into ciliated cells or neuroendocrine cells [36, 37]. Based on single cell RNAseq and marker expression studies, bipotent progenitors were identified in the mouse at the tips that express select early ATI (such as Podoplanin, PDPN) and ATII (such as Surfactant Proteins B and C, SP-B, and SP-C) markers. These are presumed to give rise to cells expressing only ATI or ATII markers just proximal to these progenitors in developing alveolar sacs (saccular stage of alveolar development , Fig. 16.1) [46, 104]. Recent studies using differentiation of hPSCs did identify cells with a phenotype compatible with bipotential alveolar progenitors, however. The pulmonary mesenchyme thins, accompanied by the development of an extensive capillary network surrounding the forming alveoli [64, 67, 68]. Subsequently, secondary septation and alveolar growth increase the surface area available to gas exchange (alveolar stage, Fig. 16.1) [64, 92]. In human most, and in rodents almost all, alveolar growth takes place postnatally [69]. During early postnatal development, lung and tracheobronchial stem cells that provide extensive regenerative capacity are laid down as well [13].
Directed Differentiation of PSCs in the Lung and Airway
Studies reporting lung differentiation from stochastically differentiating PSCs failed to show the efficiency or document depletion of other lineages [105]. Based on the paradigms established in studies on lung development in the mouse, directed differentiation of hPSCs into lung and airway cells should be accomplished by inducing definitive endoderm (DE) and subsequently specifying anterior foregut endoderm (AFE), ventral AFE, and lung field, which is then further differentiated into proximal and distal lineages.
Differentiation of PSCs into definitive endoderm. The most critical factor for DE specification in vitro is Activin A, which mimics nodal signaling in the embryo [57, 61, 62, 106, 107]. Removal of the cells from renewal conditions leads to spontaneous differentiation. In non-adherent culture conditions, the cells will form the so-called embryoid bodies, spheres of cells where spontaneous gastrulation takes place [1]. Primitive streak formation is induced by agonizing BMP4 and canonical Wnt signaling for the first 24 h. Although Wnt signaling is required for primitive streak induction [70, 106], it is often not included in differentiation protocols [76, 108, 109]. Endogenous Wnt signaling in the cultures may be sufficient for DE induction in the presence of high concentrations of Activin A. Use of an inhibitor (Y-27632) of the Rho kinase, ROCK, increases the yield of DE cells during the first day of differentiation by maintaining viability of dissociated hPSCs [110, 111]. Subsequently, the cells are exposed to high concentrations of Activin A, inducing the DE surface markers EPCAM [72], c-KIT [57], and CXCR4 [61] and the transcription factors Sox17 and Foxa2 [56, 112]. Low concentrations of BMP4 and FGF2 have also been shown to enhance DE formation in EBs [108]. However, high BMP signaling in the absence of FGF signaling promotes primitive ectoderm and trophectoderm formation [61], while BMP exposure after endoderm induction induces a hepatic fate [57]. The appropriate concentrations and duration of exposure to these factors varies between laboratories [76, 108, 109, 111] and needs to be determined empirically. Small molecules, such as two putative HDAC inhibitors, Ide1 and Ide2, may be a potential alternative to Activin A [113], although this approach has not been confirmed. The strategy used to specify DE may determine its ultimate differentiation potential. For example, we observed that lung potential arises relatively early in DE induction (d4-4.5), and is lost later on, despite continued expression of DE markers [114]. Up to 95 % pure DE can be achieved in hPSCs, although significant variability exists among lines in their capacity to generate DE. Yields appear invariably lower in mPSCs.
Anteroposterior patterning and induction of anterior foregut endoderm from PSCs. Proper specification of DE to an AFE fate is critical to generate lung field progenitors. DE generated using the strategy described above will adopt a more posterior fate [76, 115], likely explaining why the generation of lung and airway epithelial cells has lagged behind the success in the generation of pancreatic, hepatic, and intestinal cells [1, 57, 106, 116, 117]. A morphogen screen showed that exposure of human DE to a combination of BMP and TGF-β inhibition (BMPi/TGFi) led to expression of the foregut marker SOX2, suppression of the posterior marker CDX2, and maintenance of the endoderm marker FOXA2 [76], indicative of AFE specification. Timing proved to be essential, however. Application of BMPi/TGFi for longer than 48 h made the cells unresponsive to the subsequent induction of lung progenitors [76]. These findings illustrate how during development cells are appropriately receptive to signaling inputs during narrow windows of time.
It is possible that the requirement for BMPi/TGFi is explained by the dynamics of early DE development . The AFE is derived from cells that move through the primitive streak first, and then leave the node [71]. Hence, this part of the DE is the farthest removed from the nodal signaling area of the epiblast for the longest time [118]. Furthermore, these cells are exposed to the nodal inhibitor, Lefty, expressed in the visceral endoderm that surrounds the epiblast [119, 120]. During its anterior migration, the cells fated to become AFE also pass through a zone where the BMP4 inhibitor Noggin is expressed [121]. This may explain why blocking BMP and TGF-β signaling after a period of exposure to Activin A is required to specify this part of the DE. This strategy to generate AFE has been reproduced in several reports [26, 122–124]. A variation on this protocol was reported by Firth et al., where after initial BMPi/TGFi for 2 days, TGFi in the presence of BMP4 was applied for an additional 3 days [26]. Mou et al. [115] suggested that in monolayer differentiation of mouse ESCs, only inhibition of TGF-β was required for anterior patterning. The efficiency of subsequent differentiation into lung progenitors was very low, however.
Further work showed that sequential inhibition of BMP and TGF-β followed by inhibition of TGF-β and Wnt signaling over the course of 48 h improved the competence of the cells to subsequently generate lung field progenitors [114]. Indeed, in the mouse embryo, DE cells fated to become AFE pass through a zone where they are exposed to the Wnt inhibitor, Dkk1 [121], after being exposed to area where Nodal/Activin inhibitor Lefty and the BMP4 inhibitor Noggin are expressed [125, 126].
Wong et al. [25] generated AFE from hiPSCs by exposing DE to SHH and FGF2. Huang et al. however found that AFE induction using SHH and FGF2 was less efficient than using sequential BMPi/TGFi and WNTi/TGFi [114]. Clearly, the two protocols provide alternative strategies to induce AFE, suggesting the existence of complex and hierarchical relationships between TGF-β, BMP, Wnt, SHH, and FGF2 signaling in the specification of AFE from DE that warrant further study.
Specification of PSC–derived anterior foregut into ventral anterior foregut and lung field. The next step is the induction of ventral AFE and lung field. Green et al. [76] and Huang et al. [114] accomplished this in hPSCs by adding factors that are essential for lung field specification in the mouse: Wnt (or the small molecule CHIR), BMP4, FGF7, FGF10, and RA [80, 89–91, 98, 127, 128]. These manipulations resulted in cultures where, depending on the hPSCs line, up to 90 % of the cells express FOXA2+ and NKX2.1+ between days 15 and 25 [114]. The efficiency of this differentiation protocol varied across hPSC lines, a reflection of the well-documented variability in the lineage-specific differentiation potential of ES [129] and iPS [130, 131] lines. Furthermore, morphogen concentration and timing of culture stages may require optimization for each individual line. This notion is supported by the observation that ES lines are heterogeneous with respect to endogenous Wnt signaling [132]. Blocking RA, Wnt, or BMP signaling entirely abolished the generation of NKX2.1+ cells, while FGF signaling appeared dispensable in this model [114]. It is interesting to note that in vivo in the mouse model, the effect of RA is explained by inhibition of Dkk1, a Wnt inhibitor, and alleviation of TGF-β-mediated inhibition of FGF10 expression, suggesting a primarily indirect effect [89]. As in hPSCs, RA appears required even in the presence of BMP4, Wnt agonism, and FGF10; these findings suggest a direct effect of RA on lung field specification in humans [114]. These cells could be transplanted under the kidney capsule of immunodeficient mice where they gave rise to macroscopic growths, which contained cystic and tubular structures lined by a uniformly FOXA2+NKX2.1+ epithelium that ranged from pseudostratified containing cells consistent with basal, ciliated, club, and goblet cells to a monolayer consisting of flatter cells expressing markers of ATI and ATII cells. Glandular structures resembling submucosal glands were also present. No other endodermal elements were observed. However, the growths also contained smooth muscle, cartilage, and areas containing looser connective tissue, which were of human origin.
Using a ventralization strategy similar to Huang et al. [114], Gotoh et al. [123] generated human lung progenitors with similar efficiency and identified a surface marker specific for NKX2.1+ lung cells, carboxypeptidase M (CPM), which allows further flow cytometric purification. In human fetal lung, CPM was found in cells expressing ATII and ATI markers.
Mou et al. [115] induced lung field endoderm after generation of human AFE using only TGF-β inhibition using BMP4, FGF2, and Wnt. This modification produced NKX2.1+ pulmonary epithelial cells with an efficiency of 15 % in human and 10 % in mouse pluripotent stem cells , a minority of which could be induced to express proximal (p63, Sox2) markers through BMP7 agonism. Similar maturation studies in the CF iPSCs failed to show any mature cell types in vitro or in vivo. The low efficiency of lung progenitor differentiation is likely explained by suboptimal generation of AFE in the presence of only TGFi and the absence of RA in the ventralization cocktail.
Wong et al. [25], who used SHH and FGF2 to induce AFE, matured these cells towards putative lung and airway progenitors by sequential exposure to FGF7, FGF10, and BMP4 followed by FGF7, FGF10, and FGF18. The efficiency of this approach is unclear, as no immunofluorescence documentation of NKX2.1 and FOXA2 expression was provided [25]. Analysis of mRNA expression suggested some bias towards cells expressing markers of proximal airway, likely because of the presence of FGF18, which has been reported to enhance proximal and inhibit distal differentiation programs [133]. Finally, Ghaedi et al. [124] employed a variety of differentiation strategies throughout that require serum supplementation [124]. Defined conditions in the absence of serum as differentiation inducer are currently the standard of the field. This protocol [124] furthermore lacks addition of BMP4 and RA (which may be present in the serum, however), while the Wong et al. protocol lacks Wnt agonism during lung field induction [25], which we have shown to be indispensable [114], a finding that is entirely consistent with studies in mouse lung specification [64, 90, 91].
In the mouse, Longmire et al. [122] applied the protocol of Green et al. [76] with slight modifications to ESCs with a genetic reporter for pulmonary epithelium (Nkx2.1:GFP) [122]. In contrast to the human system, ventralization appeared to require FGF2, a factor that was also used by Wong et al. in human iPSCs to induce human AFE [25]. As the efficiency (as evidenced by expression of Nkx2.1:GFP) was only approximately 20 %, cell sorting was required to isolate the cells for further studies. In contrast to data in human PSCs, some of the Nkx2.1:GFP+ cells showed evidence of thyroid specification, indicated by expression of Pax8 [122]. Evident thyroid development was never observed in studies using hPSCs.
Differentiation of PSC–derived lung progenitor into more mature cell types. Terminal differentiation into mature lung and airway typically involves further culture of the cells in conditions that favor either proximal or distal differentiation.
Green et al. showed that removing BMP-4 from the ventralization cocktail (Wnt, BMP4, FGF10, FG7, RA) induced expression of SPC, a marker of ATII cells, and of early lung progenitors at E11 in the mouse (Fig. 16.2) [76]. This finding was somewhat surprising, as BMP4 has been shown to promote a more distal fate in the developing lung [85]. Consistent with these findings, however, Wong et al. showed that low concentrations of BMP4 induced proximal markers [25]. Similarly, Firth et al. found that low concentrations of BMP4 induced proximal markers, although distal markers were not assessed [26]. Finally, Huang et al. [114], also found that BMP4 addition during the terminal stages reduced expression of distal markers such as surfactant proteins and ATI markers PDPN and AQP5. They furthermore observed that continued exposure to RA also reduced distal gene expression, a finding in accordance with data from mouse genetic models. Indeed, after initiation of branching morphogenesis in mouse embryos, RA signaling inhibits distal lung and favors proximal airway development [102, 127].
Generation of proximal airway epithelium is typically accomplished by culture of lung and airway progenitors in air–liquid interphase conditions. The efficiency of the published protocols is unclear, and it is possible that this culture system is selective for those cells with proximal airway potential. Wong et al. applied ALI conditions to AFE cells further differentiated in the presence of FGF7, 10 and 18, which yielded CFTR expressing cells. Applying this strategy to iPSCs from CF patients with the F508del mutation, they could show that CFTR did not localize to the plasma membrane, as expected. After addition of a small “corrector” molecule analogue of a drug that is currently clinically used, some restoration of surface membrane localization was achieved [25]. Somewhat surprisingly, they observed expression of NKX2.1 in these presumed proximal airway epithelia. In mature lungs, however, expression of NKX2.1 is limited to ATII and club cells. Of interest is that Firth et al. [26] showed that differentiation can be biased towards ciliated cells by inhibiting Notch signaling using a γ-secretase inhibitor. Notch favors a secretory cell fate in the proximal airway epithelium by silencing the ciliated cell genetic program [36–38]. Notch inhibition, however, has also been reported to favor a distal over a proximal fate in the mouse lung in vivo [103]. The role of manipulation of Notch signaling in the differentiation of PSCs into lung and airway therefore merits further study.
Mouse ESC-derived Nkx2.1:GFP+ cells could be further differentiated in the presence of FGF2 and FGF10 and expressed a variety of airway and alveolar markers [122]. Furthermore, addition of maturation components consisting of dexamethasone, butyrylcAMP, and isobutylmethylxanthine (DCI) [134] further upregulated the expression of SP-C, SP-B, and CC-10 [122]. Nkx2.1:GFP+ cells could home to decellularized lung matrix and in some cases generate cells with marker expression and morphology consistent with ATI cells. While this work clearly showed that it is possible to differentiate PSCs into several lineages of lung and airway epithelial cells, the fact that a Nkx2.1:GFP reporter has to be used to purify cells specified to a lung field is a limitation.
Reliable distal differentiation was achieved by Huang et al. [114, 135] By continued culture in the presence of FGF7, FGF10, and Wnt agonism in addition to DCI, they achieved cultures where >50 % of the cells expressed the ATII marker, SP-B. Furthermore, after seeding onto human decellularized lung matrix, ample expression of SP-C was observed. Functionality of the cells was verified by their capacity to take up and release fluorescent SP-B. Further examination of the cultures in the long term (>50 days) revealed areas of proliferation, where cells express the ATII marker, SP-B or co-express SP-B and the ATI marker, podoplanin (PDPN) [135], suggestive of bipotential alveolar progenitors [46].
Alternative Methods
Direct reprogramming by transduction with transcription factors that drive differentiation of specific lineages is a potential alternative to directed differentiation . Direct reprogramming of ESCs has been reported for thyroid in the mouse model [136]. More often, this approach is applied to somatic cells and has met with some success for neurons [137–140], hepatocytes [141–143], cardiac myocytes [144, 145], hematopoietic stem cells [146, 147], and thymus [148] among others, but may not be useful in the lung , given the plethora of cell types required to build a functional lung and airway epithelium . Furthermore, detailed genomic analysis has revealed that cells resulting from direct reprogramming differ significantly from their normal counterparts [149]. Finally, for several organs, such as heart, reprogramming using human cells was less efficient and incomplete [150], while in others such as thymus and hematopoietic cells, no data on human cells are available yet.
A third approach is the creation of ES lines stably expressing a selectable marker driven by regulatory regions of a gene specifically expressed in the mature cell of choice. In the lung arena, the neomycin resistance gene was expressed under the control of the promoter of the ATII marker, Sftpc (SP-C), followed by selection for neomycin-resistant cells from randomly differentiating ES cells [151]. However, genetic modification and selection carries the risk of mutations in the resulting cells. Furthermore, this approach only yields one cell type, of which the functional characteristics are uncertain, with low quantitative efficiency.
Conclusion
Directed differentiation of PSCs into lung and airway epithelial cells requires discrete steps that recapitulate development as much as possible. Among published papers, there is a broad consensus on the role of Activin A in DE specification, on the use of BMPi/TGFi for anterior foregut specification. For the efficient induction of cells equivalent to the lung field, current evidence suggests that agonism of Wnt, BMP4, and RA signaling is essential. In terms of terminal differentiation, it appears that proximal and distal fates are to some extent mutually exclusive and strongly dependent on the presence of appropriate drivers of proximodistal differentiation, culture conditions, and, for distal differentiation, on the presence of physiological maturation components such as glucocorticoids.
The generation of 3D lung organoids would be a major advance. This has not been achieved yet, however. Development of 3D broncholalveolar organoids would be a first step towards this goal. 3D organoid cultures are revolutionizing the study of human development . The lung, which has lagged behind other organs such as pituitary [152], liver [153], kidney [154, 155], brain [156], eye [157], and intestine [59] in this respect [9], offers a major opportunity, as the lung consists of multiple, repeated broncho-alveolar units. Availability of such technology would also allow addressing the role of extracellular matrix in lung development and in directed differentiation of PSCs into lung and airway .
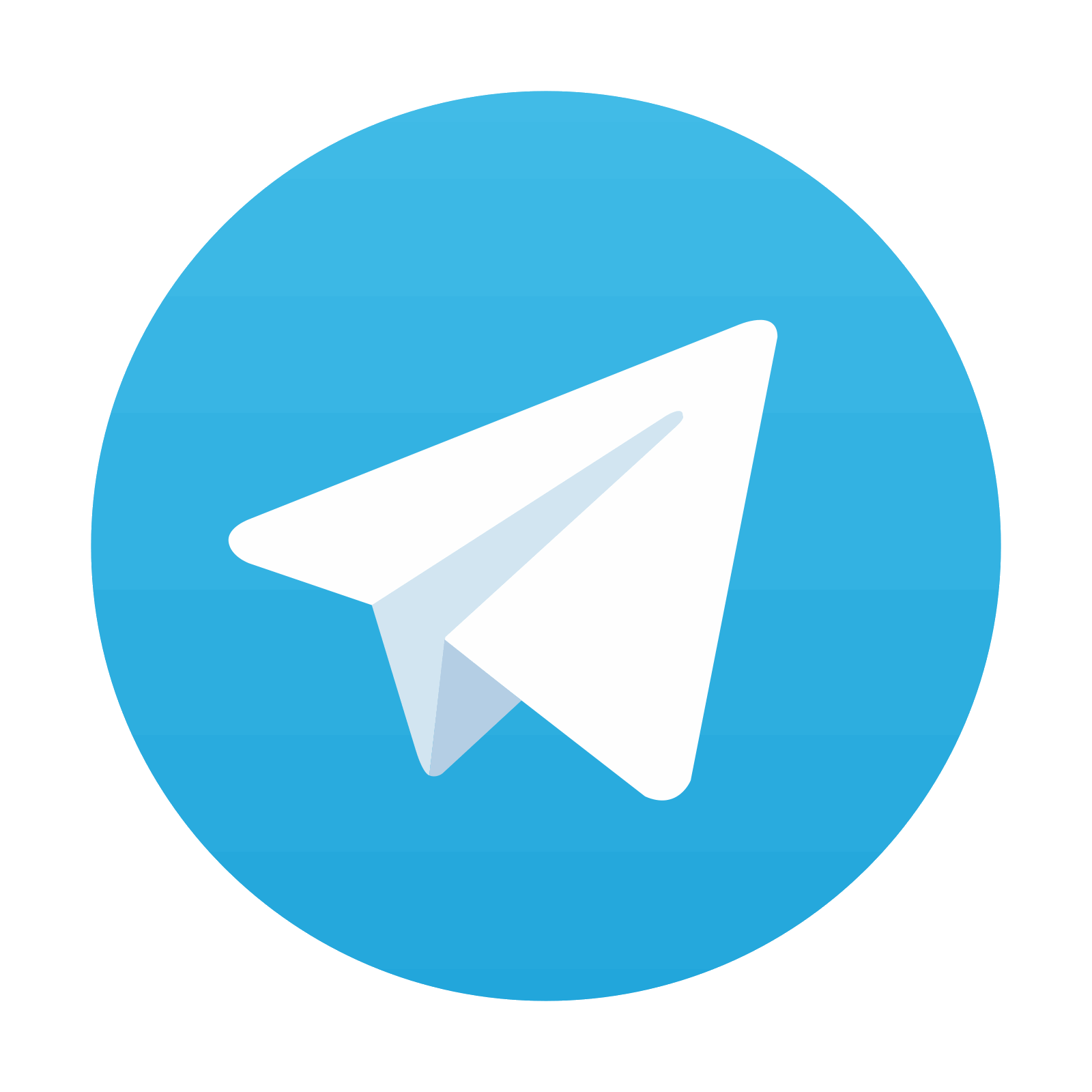
Stay updated, free articles. Join our Telegram channel
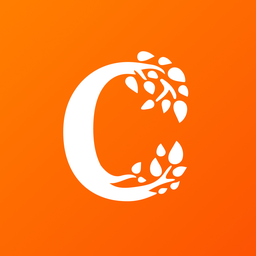
Full access? Get Clinical Tree
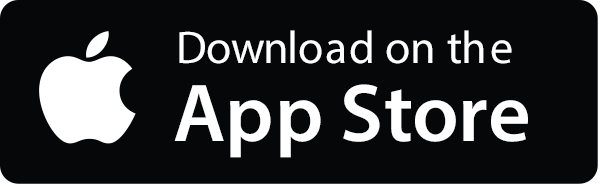
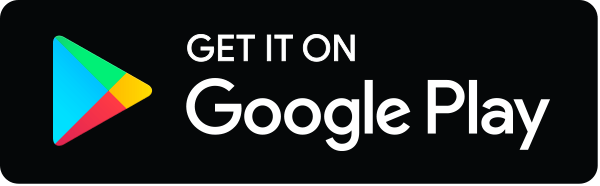