Coronary Intravascular Imaging: Introduction
Intravascular imaging continues to play a vital role in advancing our understanding of the pathophysiology of coronary artery disease (CAD) and in the development of novel cardiovascular drugs1,2 and device therapies.3,4 Gray-scale intravascular ultrasound (IVUS) was introduced more than 20 years ago and has been an integral component of the clinical decision-making process in the catheterization laboratory. Contemporary percutaneous coronary intervention (PCI) techniques mostly derived from initial IVUS observations showing that high-pressure balloon inflation was required to adequately appose metallic stents to the vessel wall and prevent thrombosis.5 Intravascular imaging has also evolved with time. IVUS technologies have added features such as tissue characterization by means of radiofrequency data (RFD) analysis to facilitate evaluation of the atherosclerotic plaque. This technique allows a more sophisticated assessment of changes in plaque characteristics over time beyond simplistic measurements of plaque dimensions.6 However, the relative poor image resolution of IVUS and physical limitations of sound have hampered a broader clinical and research application of intravascular imaging. The recent introduction of infrared light-based imaging technologies such as optical coherence tomography (OCT), which offers image resolution 10 times that of IVUS, has allowed interventional cardiologists to explore the vascular microenvironment for the first time and is expected to fuel a new wave of device, drug, and technique developments. These intravascular imaging technologies and their clinical and research applications are discussed in more detail below.
Rationale for Intravascular Imaging
Coronary x-ray angiography has retained the gold standard status in coronary imaging for more than 4 decades. Angiography depicts arteries as a planar silhouette of the contrast-filled lumen and provides a quick roadmap of the coronary circulation. Importantly, angiography does not provide visualization of the vessel wall and is not suitable for assessment of atherosclerosis. Essentially, angiography provides assessment of lumen dimensions and is used to localize and estimate the severity of obstructive coronary disease. Angiographic disease assessment is based on the comparison of the stenotic segment with the adjacent, “normal-appearing“ coronary, which is often an incorrect assumption because of the diffuse nature of atherosclerosis as shown by pathologic and IVUS studies.7 Angiography image interpretation is flawed by large inter- and intraobserver variability and usually underestimates the severity of the disease and vessel dimensions. Although quantitative coronary angiography (QCA) has reduced visual errors, the ability of arteries to enlarge to compensate for plaque growth makes angiography an unreliable method of assessing atherosclerosis burden.8 Vessel foreshortening and overlapping side branches, particularly in disease involving bifurcations, are important practical limitations of angiography and QCA. Despite the use of multiple views, characterization of eccentric lesions by angiography remains a challenge. Finally, angiographic assessment of balloon angioplasty results is hampered by the presence of contrast filled dissection and intra-plaque channels, and determination of proper stent apposition is not possible. Three-dimensional angiography at the time of the contrast injection may provide an accurate and precise assessment of the luminogram. This may address some of the limitations of conventional angiography but still does not allow visualization of the arterial wall and plaque burden. These limitations of angiography can be minimized by the intravascular tomographic visualization of lumen and vessel wall architecture. Hence, intravascular imaging plays an essential complementary role in the catheterization laboratory, enabling appropriate assessment of ambiguous disease, of vessels with aneurysmal dilatation, ostial stenoses, or disease located at branching points or in the left main (LM); tortuous or calcified segments; and eccentric plaque, complex disease morphology, intraluminal filling defects, thrombus, dissection, and lumen dimensions after coronary intervention.
Imaging Technologies
The final graphic image display is a result of reflected ultrasound waves that are converted to electrical signals and sent to an external processing system for amplification, filtering, and scan conversion. After leaving the transducer, the beam remains parallel for a short distance (near field; better image quality) and then begins to diverge (far field). The length of the near field is expressed by the equation L = r2/λ, where L is the length of the near field, r is the radius of the transducer, and λ is the wavelength. After encountering a transition between different materials—for example, the interface between blood and the intimal arterial layer—the beam will be partially reflected and partially transmitted, depending on tissue composition and differences in mechanical impedance between materials. Calcium produces nearly complete backscattering of the signal and is displayed as a bright image with a characteristic with acoustic shadowing. Ultimately, gray-scale IVUS imaging is formed by the envelope (amplitude) of the radiofrequency signal (Fig. 20–1).
Figure 20–1
The ultrasound signal is generated in a piezoelectric crystal (*) that transmits and receives sound waves (A). Ultrasound reflected by the tissue deforms crystal generating radiofrequency (RF) signal (B). Gray-scale IVUS is derived from the amplitude of RF signal, discarding information beneath the peaks of the signal. (C). Changes in the electric field of the piezoelectric crystal caused by ultrasound reflection is used to generate a gray-tone image (D). Intravascular ultrasound (IVUS) RF analysis uses several additional spectral parameters to identify four plaque components (E). Plaque components that are identified are dense calcium (white), fibrous (green), fibrofatty (greenish-yellow), and necrotic core (red) (F). IVUS palpography takes advantage of RF signals generated by the artery being deformed by blood pressure (BP). Using analysis of RF signals at “low“ and “high“ BP, the strain (deformation) in the inner layer of atheroma is determined (G). This strain is quantified and superimposed on the IVUS image at the lumen–vessel wall boundary (H). Note that high strain (yellow) is found at the shoulders of the eccentric plaque.
The quality of ultrasound images can be described by spatial resolution and contrast resolution. Spatial resolution is the ability to discriminate small objects and is determined in axial (parallel to the beam, primarily a function of wavelength) or lateral (perpendicular to the beam, a function of wavelength and aperture). Axial resolution is approximately 100 μm, and lateral resolution reaches 200 to 250 μm in conventional IVUS system (20-40 MHz) (Table 20-1). Contrast resolution is the distribution of the gray scale of the reflected signal and is often referred to as dynamic range. An image of low dynamic range appears as black and white with a few levels of gray; images at high dynamic range are often softer.
Specifications | TD-OCT | FD-OCT | IVUS |
---|---|---|---|
Axial scan/second | 5,000-10,000 | ~100,000 | |
Lines/frame | ~200 | ~500-1000 | |
Maximum frame rate, fps | 20 | ~200 | 30 |
Maximum pullback speed mm/s | 3 | 20 | 1 |
Scan diameter (FOV), mm | 6.8 | ~6-10 | 8-10 |
Axial resolution, μm | 15 | 10-15 | 100-150 |
Lateral resolution, μm | 90 | 20-40 | 200-250 |
Tissue penetration, mm | 1.5-3.0 | 2.0-3.5 | 4-8 |
Balloon occlusion | Highly recommended | No | No |
Coronary infusion required | Yes; Ringer’s lactate with occlusion balloon | Yes; iodine contrast | No |
The IVUS equipment consists of a catheter incorporating a miniaturized transducer and a console to reconstruct and display the image (Fig. 20–2). Lately, IVUS consoles have been incorporated into the catheterization laboratory equipment for easier operation. Current catheters range from 2.6 to 3.2 French (Fr) in size and can be introduced through conventional 6-Fr guide catheters. Rotational, mechanical IVUS catheters operate at frequencies between 30 and 40 MHz, and electronic phased-array systems operate at a center frequency of approximately 20 MHz. Higher ultrasound frequencies are associated with better image resolution. At 30 MHz, the wavelength is 50 μm, yielding a practical axial resolution of approximately 150 μm. But increasing the frequency beyond 40 MHz has been limited because of decreased tissue penetration.9,10 The mechanical probes rotate a single piezoelectric transducer at 1800 rpm, yielding 30 images per second. Electronic systems have up to 64 transducer elements in an annular array that are activated sequentially to generate the cross-sectional image.10 In general, whereas electronic catheter designs are slightly easier to set up and use, mechanical probes offer superior image quality. Images may be recorded on videotape or digitally with both systems. Electronic IVUS catheters have the ability to display blood flow in color to facilitate distinction between lumen and wall boundaries.
More recently, autoregressive spectral analysis of IVUS backscattered data has been incorporated into conventional IVUS systems to facilitate image interpretation of different tissue components (see Fig. 20–1). This function displays different plaque components in a color-coded scheme according to tissue signal properties (necrotic core in red, dense calcium in white, fibrous in dark green, and fibrofatty in light green).11 In a postmortem validation study, radiofrequency analysis demonstrated sensitivity and specificity for detection of necrotic core of 92% and 97%, respectively.11 The initial algorithm was validated using the mechanical rotating single-element IVUS system, but the first commercially available IVUS backscattering image analysis, named virtual histology (IVUS-VH), was built on the electronic 20-MHz IVUS platform. This system has been used in clinical trials to monitor progression of atherosclerosis. Lately, rotational mechanical IVUS systems have also integrated a backscattering regression algorithm.
Another approach is to assess the deformability of coronary plaque using the analysis of radiofrequency signals at different diastolic pressure levels, normalized to a pressure difference of 2.5 mm Hg per frame. This allows the construction of a “strain“ image, in which harder (low strain) and softer (high strain) regions of the coronary arteries can be identified, with radial strain values ranging between 0% and 2%12 (see Fig. 20–1). Postmortem coronary arteries were investigated with histology and IVUS palpography. The sensitivity and specificity of palpography to detect vulnerable plaques are 88%, and 89%, respectively.12
The IVUS procedure is performed using standard guide catheters (≥6 Fr) under full anticoagulation with an activated clotting time of more than 250 seconds. After intracoronary infusion of intracoronary nitroglycerine (100-200 μg) to minimize vasospasm, the rapid-exchange IVUS catheters are introduced in the coronary over a standard 0.014-in guidewire. Mechanical IVUS systems require infusion of heparinized saline through a dedicated port to clear air bubbles inside the sheath covering the transducer before inserting the catheter in the guide catheter. The IVUS catheter should be advanced under fluoroscopy guidance approximately 10 mm distal to the segment of interest. The operator should realize that the distal marker in mechanical systems at the tip of the catheter is a radiopaque marker and not the ultrasound probe. If possible, the catheter should be advanced beyond 10 mm and retracted slowly to straighten the catheter shaft, which may have built some slack during insertion, to minimize nonuniform rotation distortion (NURD) artifacts. Motorized pullback devices should be used to withdraw the catheter at a constant speed (most frequently at 0.5 mm/s) to allow proper examination of the entire coronary and calculation of distances. During pullback, the record function should be activated to allow storage of images with concomitant voice narration of interesting findings for offline detailed analysis. Unless coronary ischemia ensues, the catheter should be withdrawn up to the aortic coronary junction and the guide catheter should be retracted slightly to allow imaging of the coronary ostium. Side branches visualized by angiography or ultrasonography are useful landmarks to facilitate interpretation and comparisons in sequential examinations.
IVUS has been performed safely in a large number of subjects enrolled in research studies with no apparent increase in the incidence of adverse effects. The rate of complications associated with IVUS images was investigated in 2207 patients from 28 centers (including 915 patients studied for diagnostic purposes).13 A total of 87 patients (3.9%) had complications judged to be unrelated to IVUS imaging, and 63 patients (2.9%) had transient vasospasm. In nine patients (0.4%), complications were judged to be related to the IVUS procedure, including five acute vessel occlusions, three dissections, and one embolism. In 14 patients (0.6%), complications were judged to have an “uncertain“ relationship to IVUS. Major events (acute myocardial infarction [MI] or emergency bypass surgery) occurred in 3 of 9 and 5 of 14 of these patients, respectively. The complication rate was higher in patients with unstable angina or acute MI and in patients undergoing intervention compared with diagnostic IVUS.
Another report comprising 718 IVUS “examinations“ performed at 12 centers reported a 1.1% rate of complications without adverse clinical consequences. There were four cases of transient vasospasm, two cases of dissection, and two entrapment of the guidewire. All complications occurred in patients with unstable angina undergoing PCI. In 7085 IVUS studies from 51 centers,14 vasospasm occurred in 3% of patients. Major complications (dissection, thrombosis, ventricular fibrillation, and refractory spasm) occurred in 10 (0.14%). There was only one major event.
The long-term safety of IVUS in transplant recipients has also been reported.15 Overall, 548 coronary arteries in 226 patients were imaged by IVUS and compared with 130 arteries not assessed by IVUS. Subsequent angiographic stenoses were observed in 19.5% (107 of 548) of imaged arteries versus 16.2% (21 of 130) of nonimaged arteries (P = .4). Another study16 reported 18- to 24-month quantitative coronary angiographic analysis comparing IVUS-imaged and non–IVUS-imaged arteries in 525 patients. New stenoses lesions occurred in 3.6% and 3.9% of IVUS-imaged and non–IVUS-imaged arteries, respectively (P = .84). When all coronary lesions were considered, the incidence of lesion progression was not significantly different between IVUS-imaged (11.6%) and non-IVUS-imaged (9.8%) arteries. Coronary spasm occurred in 1.9% of IVUS procedures, and there was one case of acute occlusion with no long-term sequelae.
The echogenicity and texture of different tissue components may exhibit comparable acoustic properties. The similar appearance of different materials represents an inherent limitation of all gray-scale IVUS systems. For example, an echolucent intraluminal image may represent thrombus, atheroma with a high lipid content, retained contrast, or an air bubble.
Most mechanical limitations of IVUS imaging are specific to the construct of each system. NURD is specific to mechanical catheters and arises from friction of the transducer in the coronary or guiding catheter or from a poor connection of the IVUS catheter in the motor drive unit, which causes a typical “onion skin“ image appearance. Tortuosity, severely stenotic segments, small guide lumen size, guide catheters with sharp secondary curves, slack in the catheter shaft or tightened hemostatic valve are common causes of NURD. The ring-down artifact, however, is specific to electronic systems and is caused by transducer oscillations that obscure the near-field image.17Side lobes artifacts are intense reflections that come from strong reflectors such as calcium and stent struts. These usually follow the circumferential sweep of the beam. The presence of the side lobes may mask the actual lumen edge or may also be taken as tissue prolapse or dissection flaps. Reverberation is another artifact that comes from strong reflectors and is concentric repetitions at equidistant locations of the same image.
An eccentric or nonperpendicular position of the IVUS catheter produces geometric distortions and an artificially elliptical appearance of the cross-sectional image, leading to overestimation of the lumen area.17 The speed of catheter pullback is also prone to errors, which may lead to incorrect assessment of the length of the segment of interest.18 In non–sheath-based catheters (electronic system), the pitfalls of automatic pullback are greater and include the presence of catheter slack outside the patient and friction in the coronary artery and guiding catheter during pullback. Sheath-based, mechanical catheter systems allow more uniform pullbacks during image acquisition and more precise length measurements.18 The accuracy of four IVUS pullback systems was evaluated in 180 patients (45 in each group) who had been treated with a single stent of known length ranging from 8 to 33 mm. The correlation between the actual versus IVUS-measured stent lengths was 0.92 for Cardiovascular Imaging Systems (CVIS), 0.83 for Galaxy (both from Boston Scientific Corporation, IVUS Technology Center, Fremont, CA), 0.63 for Endosonics Track-Back, and 0.69 for Volcano Model R-l00 (both from Volcano Corporation, Rancho Cordova, California) pullback devices.18 The pullback speed is least accurate at the beginning of an imaging run (in the distal artery) and most accurate in the proximal artery. In battery-powered pullback devices, the battery charge should be verified at the beginning of the procedure to ensure uniform pullback speed. Ideally, the same pullback device and catheter should be used in repeated longitudinal studies. Finally, one should realize that intravascular imaging only assesses a single coronary segment at a time.
OCT evolved from optical one-dimensional, low-coherence reflectometry, which uses a Michelson interferometer and a broadband light source. The addition of transverse B-scans by James Fujimoto in 1991 enabled a two-dimensional imaging of the retina.19 Intravascular OCT uses a single optical fiber that both emits and records light reflection. The image is formed by the backscattering of light from the vessel wall based on “echo time delay“ with measurable signal intensity. The speed of light (3 × 108 m/s) is much faster than that of sound (1500 m/s), thus requiring interferometry techniques because direct signal quantification cannot be achieved on such a time scale. The interferometer uses a fiberoptic coupler similar to a beam splitter, which directs half of the beam to the tissue and the other half to the reference arm. The reference arm of a time-domain OCT system (TD-OCT) consists of a mirror that moves to produce variable, yet known echo delays. Newer generations of intravascular OCT systems use a fixed mirror with a variable frequency light source or “swept laser.“ This method, termed Fourier-domain OCT (FD-OCT), allows the simultaneous detection of reflections from all echo time delays, making the system significantly faster.20 There are two types of FD-OCT systems that differ in their method of data extraction from the interferometer: optical frequency domain imaging (OFDI), also known as swept-source OCT-(SS-OCT), and spectral domain OCT (SD-OCT). OCT images are formed by the reflected signal returning from the tissue and reference arms “interferes“ in the fiber coupler and are detected by a photodetector. The general schemes of intravascular OCT systems are shown in Fig. 20–3.
Figure 20–3
The upper panels show the first-generation commercially available time-domain optical coherence tomography (TD-OCT) imaging system console (LightLab M3), Helios™ balloon, and ImageWire™ (A), and the new-generation frequency-domain or Fourier-domain (FD) OCT imaging console (LightLab C7XR™) and imaging catheter (B). The lower panels show schematic representation of TD-OCT (C) and FD-OCT (D). Both systems use a reference arm and an interferometer to detect echo time delays of light. The interferometer uses a beamsplitter, dividing the light into a measurement arm (tissue sample) and a reference arm. The reference arm in TD-OCT is mechanically scanned (by a moving mirror) to produce a varying time delay. In the FD-OCT, the light source is frequency swept, and the interference of the two light beams (tissue and reference) oscillates according to the frequency difference. In both systems, the interference of the signal ultimately provides amplitude and frequency data. In the FD-OCT system, all echo delays are acquired simultaneously, enabling significant increases in the speed of image acquisition.
As the fiber optic rotates, multiple axial scans (A-lines) are continuously acquired to generate the cross-sectional vascular image. Bandwidths in the near-infrared spectrum with central wavelengths ranging from 1250 to 1350 nm are used for intravascular applications. Longer wavelengths would provide deeper tissue penetration but would be limited by absorption and the refractive index caused by protein, water, hemoglobin, and lipids. Thus, the image depth of current OCT systems ranges from 1 to 3 mm into the vessel wall. The axial resolution, which is determined by the light wavelength, ranges from 12 to 20 μm. The lateral resolution in catheter-based OCT is typically 20 to 90 μm (see Table 20-1).
The first clinically available OCT systems used time-domain (TD) technology (M2 and M3 OCT Imaging Systems, LightLab Imaging, Inc., Westford, MA). The newer systems are based on frequency domain technology. TD-OCT has been approved for clinical use in the European Union, Asia, Japan, and South America but is limited to research applications in North America. The system consists of a single-mode fiberoptic wire (ImageWireTM), which is able to rotate inside a fluid-filled polymer sheath. An over-the-wire low-pressure occlusion balloon catheter (Helios Goodman, Advantec Vascular Corp.TM) with distal flush ports is used to infuse saline or Ringer’s lactate at approximately 0.5 mL/s to displace blood during imaging acquisition.
The newer Lightlab C7XRTM (FD-OCT) system is approved for clinical use in most countries including the United States. It is equipped with a tunable laser light source, with the fiber encapsulated within a rotating torque wire built in a rapid exchange 2.6-Fr catheter compatible with 6-Fr guides. Terumo (Terumo Corporation, Tokyo, Japan) has also developed an FD-OCT system, which has a 2.4-Fr shaft. The CorVueTM Spectral Domain System (Volcano Corporation, Rancho Cordova, CA) is the third FD-OCT system under development with a rapid-exchange nitinol hybrid drive shaft.21
General principles of intravascular imaging also apply to OCT. Standard guide catheters (≥6 Fr) are used, patients must receive full anticoagulation, the image wire or catheter should be advanced under fluoroscopy guidance approximately 10 mm distal to the segment of interest, and the image is acquired after intracoronary infusion of intracoronary nitroglycerine. Despite its guidewire-like profile, the OCT ImageWire is not designed to be advanced into the coronary artery as a stand-alone device. Thus, a conventional angioplasty guidewire (0.014 in) should be inserted first and exchanged to the ImageWire using the Helios or a micro catheter with an inner diameter larger than 0.019 in. Proper OCT imaging requires a blood-free environment because any amount of residual red blood cells (RBCs) causes signal attenuation. In TD-OCT, this is achieved by the inflation of the occlusion balloon proximal to the segment of interest. After advancing the ImageWire into the distal coronary segment, the occlusion balloon is pulled back and repositioned in a healthy proximal segment. Before inflating the HeliosTM balloon at 0.4 to 0.7 atm using a dedicated inflator, injection of Ringer’s lactate at 0.5 cc/s should be started using an injector pump with a warming cuff. Infusion rates can be increased to up to 1.0 cc/s until blood is completely cleared. In TD-OCT systems, the pullback speed can be adjusted from 0.5 to 3.0 mm/s.
FD-OCT are built in rapid-exchange, 6-Fr compatible, catheter-based systems that are advanced into the coronary segment over a standard 0.014-in guidewire. These systems can acquire 100 to 160 image frames/s, reaching pullback speeds up to 20 to 40 mm/s with the potential to scan epicardial coronary vessels that are 4 to 6 cm long in less than 3 seconds22,23 with the use of a single, high-rate (~4 cc/s) bolus injection of contrast (see Table 20-1). For nonocclusion techniques, iodinated contrast media is preferred over saline or Ringer’s lactate because of the advantage of high-viscosity solutions in completely removing blood. Before introducing the catheter, a small amount of iodine contrast must be purged into the covering sheath to clear air bubbles and prevent blood entry. The C7 system uses a similar console and pullback system as the M3 system; the activation of the pullback is automatic as the blood is cleared from the lumen and triggers recording of images, which are stored electronically. The pullback system also has a function to re-advance the fiber within its cover sheath. The fast pullback speed does not allow voice recording during imaging acquisition of FD-OCT systems.
The OCT imaging technique has been adopted in many centers across Europe and Japan, and various centers have presented large series of patients imaged by OCT without major complications. The applied energy in intravascular OCT is relatively low (output power in the range of 5.0-8.0 mW) and is not considered to cause functional or structural damage to the tissue. Safety concerns thus seem mainly dependent on the mechanical properties and need for blood clearance for image acquisition. Studies comparing OCT with IVUS suggest that TD-OCT is safe and can be performed with success rates at least comparable with IVUS.24-26 The most frequent complication in 76 patients imaged with TD-OCT using the occlusive OCT technique were transient chest discomfort, brady- or tachycardia, and ST-T changes on electrocardiography (ECG), all of which resolved immediately after the procedure. Similar transient events were also seen during IVUS imaging procedures. Neither hemodynamic instability nor ventricular tachyarrhythmia was observed.25 In addition, in a multicenter registry, the acute complications associated with OCT were assessed in 468 patients. TD-OCT was performed using a non-occlusive flush technique in 45.3% of the patients with a mean contrast volume of 36.6 ± 9.4 mL (M2 and M3). Transient chest pain and QRS widening or ST-depression or elevation were observed in 47.6% and 45.5% of patients, respectively. Major complications were rare; they included five (1.1%) cases of ventricular fibrillation caused by balloon occlusion or deep guide catheter intubation, three (0.6%) cases of air embolism, and one (0.2%) case of vessel dissection. There were no cases of coronary spasm or major cardiovascular events (MACE) during or within the 24-hour period after OCT examination.27 The more recent nonocclusive FD-OCT technique (C7XR) has led to an important reduction in the image acquisition time (3-4 seconds) and in the rate of chest pain and ECG changes during image acquisition, but the feasibility and safety of the larger catheter-based FD-OCT systems remain to be established in future trials.
The need for a proximally placed balloon in conventional TD-OCT imaging limits its ability to evaluate proximal coronary segments and ostial disease. Operators should be aware of the vessel size range suitable for TD-OCT imaging, which has a field of view between 2.0 to 3.75 mm in diameter. “Out-of-screen“ loss of image represents the most common technical failure in OCT imaging. FD-OCT has a much larger filed of view (see Table 20–1).
Some image artifacts are common to both OCT and IVUS. NURD can result in focal image loss or shape distortion but seems to occur less frequently in OCT than in IVUS imaging, perhaps as a result of the smaller profile and simplified rotational mechanics of OCT wires. Sew-up artifact is the result of rapid artery or imaging wire movement in one frame’s imaging formation, leading to misalignment of the lumen border. Eccentricity intraluminal position of the ImageWire may lead to wider distances between A-lines and consequently decrease lateral resolution. This effect has been dubbed the “merry-go-round.“ Similarly, one can notice the reflection from metallic stent struts aligning toward an eccentrically positioned ImageWire (“ sunflower“ effect). The saturation artifact occurs when light reflected from a highly specular surface (usually stent struts) produces signals with amplitudes that exceed the dynamic range of the data acquisition system. While these artifacts are common to all intravascular imaging technologies, others are specific to OCT imaging. Residual blood produces light attenuation and may defocus the beam if the RBC density is high. This reduces the brightness of the vessel wall. Residual blood does not appear to affect area measurements in OCT images with a clearly defined lumen–wall interface. The operator should be aware that residual blood can mimic the OCT appearance of thrombus. Fold-over artifact is specific to the new generation of FD-OCT systems and is the consequence of the “phase wrapping“ or “alias“ along the Fourier transformation when structure signals are reflected beyond the field of view. This can occur at the site of bifurcations or in large vessels. Air bubbles can form within the silicon lubricant used to reduce friction between the sheath and the optic fiber in TD-OCT systems, causing light attenuation (Fig. 20–4).
Figure 20–4
Cross-sectional optical coherence tomography images of human coronary arteries depicting the most frequently observed artifacts: A. Vessel “out-of-screen.“ B. Sew-up artifact resulting in misalignment of the image. C. Nonuniform rotational distortion (NURD), usually produced by a tight bend or by an imperfection in the torque wire or sheath that interferes with rotation speed. D. Incomplete intracoronary blood displacement causing light attenuation. E. The presence of blood inside the image catheter sheath. F. Fold-over artifact observed in a large vessel bifurcation. G. Saturation artifact; some scan lines have a streaked appearance. H. Light attenuation caused by air bubbles within the silicon lubricant used to reduce friction between the sheath and the optic fiber in time-domain optical coherence tomography (TD-OCT) systems. I. Eccentric intraluminal location of the ImageWire can distort stent reflection, causing struts to align toward the imaging wire (“ sunflower effect“ , left box) or appear elongated (“ merry-go-round“ , right box).
Another imaging modality able to detect necrotic core invasively is near-infrared NIR spectroscopy (NIRS).28 To this aim, the 3.2-Fr FDA-approved NIRS catheter is used. This catheter is compatible with a conventional 0.014-in guidewire, contains a rotating (240-Hz) NIRS light source at its tip, and is pulled back by a motor drive unit at 0.5 mm/s. The catheter’s ability to detect lipid core plaques (LCP) has been validated successfully in a human autopsy study in which the device algorithm prospectively identified LCP with a receiver–operator characteristic area of 0.80 (95% confidence interval [CI], 0.76-0.85).29 The new 3.2-Fr rapid exchange Apollo catheter combines a 40-MHz real-time IVUS catheter with a frame speed of 16 frames/s operating at a pullback speed of 0.5 mm/s with a standard NIRS catheter. In the SPECTACL (SPECTroscopic Assessment of Coronary Lipid) trial, which was a parallel, first-in-human, multicenter study designed to demonstrate the safety applicability of the LCP detection algorithm in 106 living patients.30
Characterization of Atherosclerosis
Understanding of the normal structure of a normal coronary artery is essential to differentiate its pathologic conditions. The arterial wall of coronary arteries is composed of three layers. The innermost tunica intima is in direct contact with the blood and is constituted by an endothelial cell monolayer resting on a basement membrane. Aging of human arteries is associated with the presence of smooth muscle cells in the tunica intima. These cells produce extracellular matrix molecules, leading to intimal layer thickening. This process is not necessarily associated with pathologic lipid accumulation and atherosclerosis formation. The second layer, the tunica media, is separated from the tunica intima by the internal elastic membrane (IEM) and is formed by concentric layers of smooth muscle cells. The adventitia is the outermost arterial layer, which is separated from the media by the external elastic membrane (EEM) and contains fibroblasts and mast cells, collagen fibrils, vasa vasorum, and nerve endings.
The normal coronary architecture can be assessed using intravascular imaging. The circulating blood elements may assist IVUS image interpretation and differentiate the lumen from the vessel wall because these produce characteristic speckles in the image. However, appearance of blood speckles depend on the flow velocity and may have increased intensity in situation of slow blood flow, causing it to have a similar appearance as the vessel wall. The reported normal value for intimal thickness in young subjects is typically 0.15 ± 0.07 mm. Thus, the thin tunica intima poorly reflects ultrasound and is not visualized as a separate layer. The media is typically less echogenic than the intima but may appear thick because of signal attenuation and weak reflectivity of the IEM. A monolayer appearance is a common finding in normal coronary arteries, but a trilayered appearance by IVUS suggests the presence of intimal thickening.31 A trilaminar appearance depends not only on the age but also on the histologic characteristics of the vessel. The adventitia has the strongest echo signal, which is used as a reference to determine plaque components. Importantly, the IVUS beam penetrates beyond the adventitial layer, allowing visualization of perivascular structures, including the cardiac veins and the pericardium.
In contrast, the normal coronary artery wall (<1.5 mm thick) appears as a three-layer structure on OCT, but imaging beyond the adventitial layer is not possible. The normal thickness of the tunica intima is approximately 4 μm, which is beyond the resolution capacity of current OCT systems. The first visualized layer is indeed the IEL (not seen by IVUS), which despite its less than 3 μm thickness, generates a 20-μm signal-rich band. The relatively thick media (>100 μm) can be visualized easily by OCT,32 which is depicted as a low-intensity band delimited by the IEL and EEL. Whereas the EEL produces a signal-rich band, the adventitia produces a signal-rich band with heterogeneous texture on OCT images.
A number of studies have demonstrated the ability of IVUS and OCT to differentiate coronary atheroma components (Figs. 20–5 and 20–6). However, it is important to realize that in vivo intravascular imaging is fundamentally different from ex vivo histology and that specific histologic plaque components cannot be assessed by conventional clinical imaging. A detailed description of atherosclerosis development and composition is beyond the scope of this chapter. In brief, an atheroma is formed by an intricate sequence of events, not necessarily in a linear chronologic order, that involves extracellular lipid accumulation, endothelial dysfunction, leukocyte recruitment, intracellular lipid accumulation (foam cells), smooth muscle cell migration and proliferation, expansion of extracellular matrix, neoangiogenesis, tissue necrosis, and mineralization at later stages. The ultimate characteristic of an atherosclerotic plaque at any given time depends on the relative contribution of each of these features.33 Thus, the pathologic intimal thickening (PIT) is rich in proteoglycans and lipid pools, but no trace of necrotic core is seen. The earliest lesion with a necrotic core is the fibroatheroma, and this is the precursor lesion that may give rise to symptomatic heart disease. Thin-capped fibroatheroma (TCFA) is a lesion characterized by a large necrotic core containing numerous cholesterol clefts. The overlying fibrous cap is thin and rich in inflammatory cells, macrophages, and T lymphocytes with few smooth muscle cells. A cut-off value for cap thickness of less than 65 μm to define a thin cap vulnerable coronary plaque has been based on pathology studies,34 but in vivo confirmation of such a threshold is lacking.
Figure 20–5
A,B, and C are corresponding images of the same coronary plaque. By gray-scale, atherosclerotic plaque can be classified into four categories: soft, fibrous, calcified, and mixed plaques (A). Virtual histology (B) is able to detect four tissue types: necrotic core, fibrous, fibrofatty, and dense calcium. At the bottom, optical coherence tomography (C) is showing the different plaque types that can be detected with this technique.
Figure 20–6
Correlation between optical coherence tomography and histology. A. Fibrotic plaque: characterized by high signal (high backscattering) and low attenuation (deep penetration). B. Predominantly calcified plaque: calcified regions have a sharp border, low signal, and low attenuation, permitting deeper penetration. C. Lipid-rich plaque: the lipid core has a diffuse border. Light attenuation results in poor tissue penetration (in contrast to calcified regions). The overlying fibrotic cap can be readily measured; in this case, a thick cap (>200 μm) is present. Calcified region; *lipid core. Courtesy of C.Y. Xu and J.M. Schmitt, LightLab Imaging.
Based on tissue echogenicity, not necessarily histologic composition, atheromas have been classified into four categories by gray-scale IVUS: (1) soft plaque (lesion echogenicity less than the surrounding adventitia), (2) fibrous plaque (intermediate echogenicity between soft [echolucent] atheromas and highly echogenic calcified plaques), (3) calcified plaque (echogenicity higher than the adventitia with acoustic shadowing), and (4) mixed plaques (no single acoustical subtype represents >80% of the plaque)17 (see Fig. 20–5).
Virtual histology can potentially identify all of the above-mentioned plaque types.35Figure 20–7 outlines the virtual histology plaque and lesion types that are proposed based on the above pathologic data.
Figure 20–7
Plaque classification using optical coherence tomography (OCT) and intravascular ultrasound virtual histology (IVUS-VH). AIT, adaptive intimal thickening; ; CaFA, calcified fibroatheroma; CaTCFA, calcified thin cap fibroatheroma; DC, dense calcium; FA, fibroatheroma; FC, fibrocalcic; FF, fibro fatty; FT, fibrotic; NC, necrotic core; PIT, pathologic intimal thickening; TCFA, thin cap fibroatheroma.
IVUS-derived thin-cap fibroatheroma (IDTCFA) using IVUS-VH has been defined as a lesion fulfilling the following criteria in at least three consecutive frames: (1) necrotic core of 10% or more without evident overlying fibrous tissue and (2) plaque burden of 40% or more. Here, thin cap is defined as all fibrous caps < 200 microns, which is the limit of the IVUS resolution. In this study, 62% of patients had at least one IDTCFA in the interrogated vessels. Patients with acute coronary syndrome (ACS) had a significantly higher incidence of IDTCFA than stable patients (P = .018). Finally, a clear clustering pattern was seen along the coronaries, with 66.7% of all IDTCFAs located in the first 20 mm. This distribution of IDTCFAs is consistent with previous clinical studies, with a clear clustering pattern from the ostium demonstrating a nonuniform distribution of vulnerable plaques along the coronary tree.37 Rioufol et al38 reported that patients presenting with ACS had a significantly higher prevalence of plaque ruptures even in nonculprit vessels, supporting the concept of a multifocal process. Of note, the plaque burden and the mean necrotic core areas of the IDTCFAs detected by IVUS-VH were also similar to previously reported histopathologic data (55.9% vs 59.6% and 19% vs 23% respectively).39
Software has been developed to quantify the amount of necrotic core in contact with the lumen, enabling refinement of our analysis. Our current definition of an IVUS-derived TCFA is a lesion fulfilling the following criteria in at least three frames: (1) plaque burden of 40% or more, and (2) a confluent necrotic core of 10% or more in direct contact with the lumen (ie, no visible overlying tissue).40 Using this refined definition of IDTCFA in patients with ACS who underwent IVUS of all three epicardial coronaries, on average, there were two IDTCFA per patient, with half of them showing outward remodeling.40
The frequency and distribution of IDTCFA identified (n = 105) and stable angina pectoris (SAP; n = 107) in a three-vessel IVUS-VH study have been recently published by Hong et al.41 There were 2.5 ± 1.5 in ACS and 1.7 ± 1.1 in SAP TCFAs per patient (P < .001). A total of 76 patients with ACS and 58 with SAP had multiple ID-TCFA (P = .009). Presentation of ACS was the only independent predictor for multiple ID-TCFA (P = .011). A total of 83% of ID-TCFA were located within 40 mm of the coronary.
The potential value of these VH IVUS-derived plaque types in the prediction of adverse coronary events was evaluated in an international multicenter prospective study, the Providing Regional Observations to Study Predictors of Events in the Coronary Tree (PROSPECT) study. The PROSPECT trial was a multicenter, natural history study of patients with ACS. All patients underwent PCI in their culprit lesion at baseline followed by angiography and IVUS virtual histology of the three major coronary arteries. A IDTCFA with a minimum lumen area of 4 mm2 or less and a large plaque burden (≥70%) had a 17.2% likelihood of causing an event within 3 years. Interestingly, the anticipated high frequency of acute thrombotic cardiovascular events did not occur, with only a 1% rate of MI and no deaths directly attributable to nonculprit vessels over 3 years of follow-up. These results suggest that nonculprit yet obstructive coronary plaques are most likely to be associated with increasing symptoms rather than thrombotic acute events, with 8.5% of patients presenting with worsening angina and 3.3% with unstable angina.
Although a 2-mm depth might be sufficient to image the entire plaque in most diseased vessels, OCT cannot serve as a routine method to assess deeper arterial wall structures such as the adventitia. Ex vivo validations have also shown that OCT is superior to conventional and integrated backscatter IVUS for characterization of coronary atherosclerotic plaque composition.42-45 In vivo, OCT is able to identify most of the architectural features identified by IVUS and may be superior for the identification of lipid pools, calcification, and thrombus.46 A study comparing OCT with histopathology reported 41% plaque misclassification mostly caused by a combination of incomplete image penetration and the inability to distinguish calcium deposits from lipid pools.47 An ex vivo OCT investigation classified 357 diseased carotid and coronary segments as fibrous, fibrocalcific, and lipid-rich plaques. Fibrous plaques were characterized as homogeneous, signal-rich regions; fibrocalcific plaques as signal-poor regions with sharp borders; and lipid-rich plaques as signal-poor regions with diffuse borders (see Figs. 20–5 and 20–6). The comparison with histology showed a sensitivity and specificity ranging from 71% to 79% and 97% to 98% for fibrous plaques, 95% to 96% and 97% for fibrocalcific plaques, and 90% to 94% and 90% to 92% for lipid-rich plaques, respectively.48 Various studies have shown a higher prevalence of lipid-rich plaques in ACS than in patients with stable angina49 and no significant impact of diabetes status and gender on the appearance of culprit plaques on OCT images.50,51 According to histologic and IVUS examinations, the percentage of lipid-rich plaque by OCT has been found to be higher in plaques with positive remodeling.52
The presence, depth, and circumferential distribution of calcification are important factors for selecting the type of interventional device and estimating the risk of vessel dissection and perforation during PCI.53 On IVUS, calcium appears as bright echoes that obstruct the penetration of ultrasound (acoustic shadowing) (see Fig. 20–6A). IVUS detects only the leading edge of calcium and cannot determine its thickness. Thus, calcification on IVUS is usually described based on its circumferential angle (arc), longitudinal length, and depth. Calcification can be located deeper in the arterial wall or in the surface of the plaque in close contact with the lumen wall interface. As mentioned above, calcium can produce reverberations or repeated reflections at reproducible distances. IVUS has shown significantly higher sensitivity than fluoroscopy in the detection of coronary calcification.54 Virtual histology, compared with histology, has a predictive accuracy of 96.7% for detection of dense calcium.55
In contrast to ultrasonography, light penetrates calcium well, and OCT can depict calcification within plaques as a well-delineated, low-backscattering heterogeneous regions (see Figs. 20–5 and 20–6). In addition to circumferential angulation, depth, and longitudinal length, OCT can quantify calcium burden. Superficial microcalcifications can also be identified on OCT images as small calcific deposits separated from the lumen by a thin tissue layer. A sensitivity of 96% and a specificity of 97% to detect calcified nodules by OCT compared with histology have been reported.48
Arterial remodeling refers to a continuous process involving positive or negative changes in vessel size measured by the EEM cross-sectional area (CSA). Positive remodeling occurs when there is an outward increase in EEM. Negative remodeling occurs when the EEM decreases in size (shrinkage of the vessel).17 The magnitude and direction of remodeling can be expressed by following index: EEM CSA at the plaque site divided by EEM CSA at the reference “nondiseased“ vessel. Whereas positive remodeling will demonstrate an index above 1.0, negative remodeling has an index below 1.0. Direct evidence of remodeling can only be demonstrated in serial studies showing changes in the EEM CSA over time because remodeling may also be encountered at the “normal-appearing“ reference coronary segment.17
Whether this phenomenon, which was first described by Glagov,56 is an independent process or an adaptive response to compensate for plaque growth is still debated. The limitation of angiography in determining disease burden and stenosis severity is largely because of vessel remodeling. Detection of remodeling is extremely important during PCI to define plaque burden and the appropriate size of devices. The limited penetration of light into tissue poses significant limitations for appropriate assessment of remodeling by OCT.
Pathologic studies have also suggested a relationship between positive vessel remodeling and plaque vulnerability. Vessels with positive remodeling showed increased inflammatory marker concentrations, larger lipid cores, a paucity of smooth muscle cells, and medial thinning.57-59 Several IVUS studies have linked positive vessel remodeling with culprit60 and ruptured coronary plaques.61,62 Positive remodeling has been observed more often in patients with ACS than in those with stable CAD63,64 and has been identified as an independent predictor of major adverse cardiac events in patients with unstable angina.65 Plaques exhibiting positive remodeling have also shown more often thrombus and signs of rupture.66 The pattern of remodeling has also been correlated with plaque composition: whereas soft plaques are associated with positive remodeling, fibrocalcific plaques more often have negative or constrictive remodeling.67 Similar findings have been observed in studies using IVUS RFD analysis, a technique developed specifically for tissue characterization. Positive remodeling was directly correlated with the presence and size of the necrotic core and inversely associated with fibrotic tissue.68
ACS are often the first manifestation of coronary atherosclerosis, making the identification of plaques at high risk for complication an important component of strategies to reduce deaths associated with atherosclerosis. Our current understanding of plaque biology suggests that about 80% of clinically evident plaque rupture originates within an inflamed TCFA.69,70 Plaque ruptures occur at sites of significant plaque accumulation but are often not highly stenotic by coronary angiography because of positive vascular remodeling.61,62,71 The transition to plaque rupture has been characterized by the presence of active inflammation (monocyte or macrophage infiltration), thinning of the fibrous cap (<80 μm), development of large lipid necrotic core, endothelial denudation with superficial platelet aggregation, and intraplaque hemorrhage.72 The remaining plaques that cause ACS contain calcium nodules (~10%) or have none of the pathologic features described (~20%). Superficial plaque erosion explains at least a portion of the latter events, particularly in women73 and individuals with diabetes. The lack of cellular or anatomical signature of plaque erosion makes it difficult for existing imaging methods to have high accuracy in predicting ACS events. In addition, most plaque ruptures are silent without clinical manifestations, and repetitive healed ruptures may contribute to stable progression into obstructive disease.74 Although plaque characteristics do not yet influence current therapeutic guidelines, the available clinical imaging modalities, IVUS and OCT, have the ability to identify some of the pathologic atheroma features described above (see Figs. 20–5 and 20–6).
There are no definitive IVUS features that define a plaque as vulnerable, and ruptured plaques may have a variable appearance. Most commonly, IVUS may reveal an “axial,“ abrupt ulceration depicted as an echolucent “void“ or cavity beginning at the luminal–intimal border. These features should be distinguished from longitudinal tears of the intima and media associated with spontaneous or iatrogenic dissection. The tear of the rupture in the fibrous cap can be identified in approximately 60% of the cases and occurs more often at the shoulder of the plaque than in the center.61,75
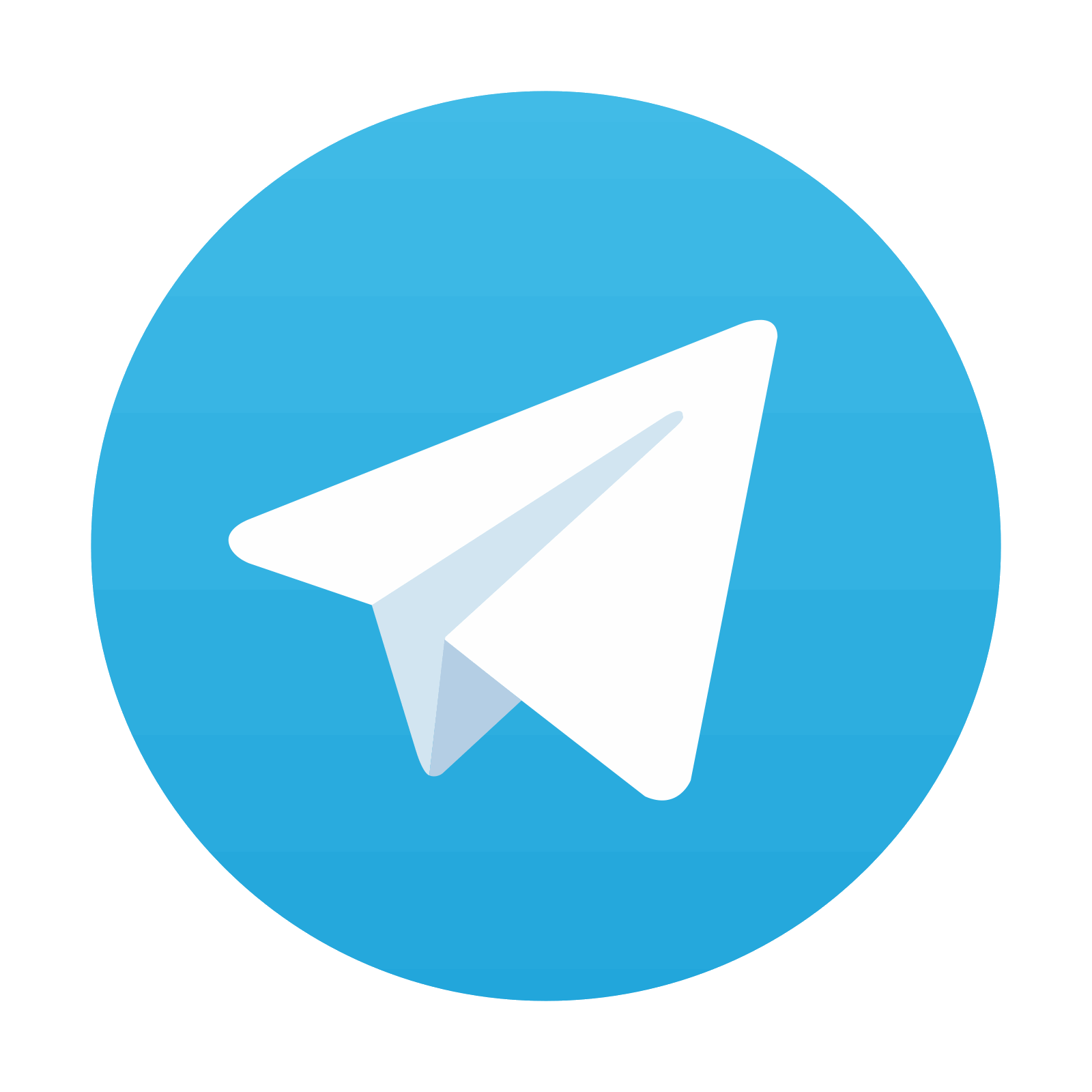
Stay updated, free articles. Join our Telegram channel
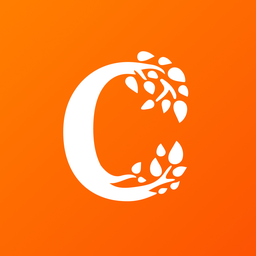
Full access? Get Clinical Tree
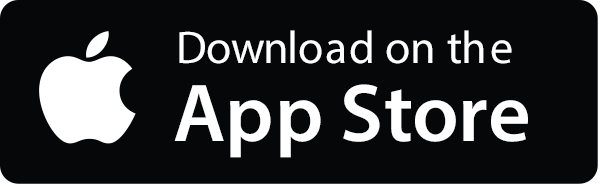
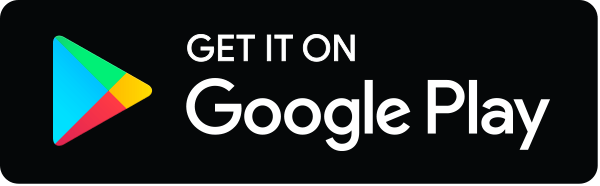
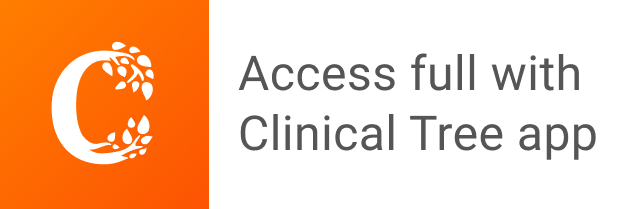