Fig. 9.1
DSA images of the femoral vein. (a) Immediately before contrast injection, an X-ray “mask” image is obtained. (b) Imaging is performed during injection of contrast into a vessel. (c) A subtraction image is created by “subtracting” the mask image from the injection image, which greatly improves visualization of the contrast-filled blood vessel
Conventional venography requires an IV or catheter to be inserted into the venous system of interest. The access site must be distal to the vein(s) of concern; for example, to image the entire venous system of the leg, the IV must be inserted into a foot vein. Iodinated contrast, which is much denser than blood, is injected into the IV or catheter. During the injection, the operator utilizes fluoroscopy to visualize and record the flow of contrast through the veins. During the study, the operator can focus on areas of interest, using various techniques to alter flow dynamics, such as the use of tourniquets, varied arm or leg positioning, and additional venipuncture sites. Furthermore, if deemed necessary, the venous access site can be used to perform endovascular interventions if deemed appropriate at that time. Since iodinated contrast can worsen renal function in patients with renal impairment, the renal function should be documented prior to performing this procedure [1]. At our institution, a serum creatinine level above 2.0 mg/dL would be a relative contraindication for conventional venography. Furthermore, there is a significant incidence of allergic reactions to contrast agents, and therefore a careful history should be performed. In cases of minor allergic reaction, such as hives, a corticosteroid premedication regimen is often used.
Conventional venography is considered to be the gold standard for venous imaging, particularly for the diagnosis of DVT and venous stenosis [2, 3]. Validation of all other imaging modalities has historically been based upon comparison to conventional venography. For imaging small veins and branches, the superior spatial resolution of conventional venography renders it superior to all other modalities [4]. Conventional venography can readily diagnose venous thrombosis and venous disease throughout the body. Because conventional venography allows visualization of the flow dynamics of the contrast bolus, collateral venous flow in the setting of venous obstruction is very well demonstrated (Fig. 9.2). This is particularly helpful for determining the hemodynamic significance of a venous stenosis and chronicity of any obstructive pathology. Furthermore, repeat imaging with altered extremity positioning (i.e., provocative maneuvers) can be utilized to recreate symptoms, such as thoracic outlet syndrome. Stenosis, reflux, incompetent valves, and reversal of flow are also well evaluated with conventional venography. Venous mapping for hemodialysis access planning is commonly performed with conventional venography, given the ability to rapidly and easily visualize the configuration of the upper extremity venous system. Another advantage of conventional venography is the ability to perform endovascular therapies at the time of diagnosis. Conventional venography can be tailored specifically for each patient with excellent flexibility for problem solving.
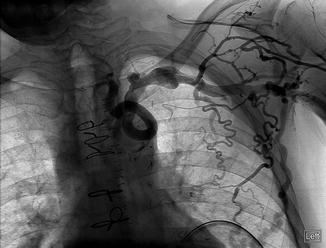
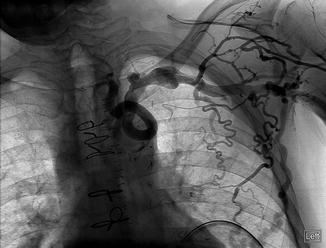
Fig. 9.2
Collateral veins are well demonstrated with conventional venography of this patient with a left brachiocephalic vein occlusion
The primary disadvantage of conventional venography is its invasive nature. Because injection of contrast into a vein results in opacification of the associated draining veins in their physiologic direction of flow (typically towards the heart), a vein in the affected extremity must be accessed as distally as possible. In patients with suspected DVT or stenosis, arm or leg swelling is the typical presenting symptom, and thus accessing veins in edematous hands and feet can be extremely challenging. Furthermore, pedal vein access in diabetic patients poses a significant infection risk. In general, there is a 2 % chance of IV or catheter-induced DVT with venipuncture [3]. Iodinated contrast is, by far, the most commonly used contrast agent for conventional venography, although, in rare circumstances, gadolinium or carbon dioxide can be used as the contrast agent. Another disadvantage of conventional venography is that visualization of an opacified vein is highly dependent upon the concentration of contrast. When imaging veins near the IV insertion site, a high concentration of contrast can be easily achieved. However, as the contrast bolus travels centrally into larger veins, progressive contrast dilution occurs, which can limit visualization of the more centrally located vein(s). This problem is markedly worsened in the setting of stenotic or occluded veins, where visualization of the vein(s) central to the lesions can be difficult to impossible when distant from the IV. Furthermore, only the veins in the direct line of flow from the IV access site to the right atrium will be opacified with contrast. This requires multiple access sites if multiple venous distributions are in need of imaging. For example, evaluation of the bilateral pelvic veins requires bilateral lower extremity IVs. A technical disadvantage of conventional venography is inflow artifact, which is the disturbance of the contrast column by non-opacified blood flowing into the opacified vein from a branch vein (Fig. 9.3). This can cause the appearance of a filling defect or stenosis. Careful inspection of a questionable filling defect on sequential frames should demonstrate some degree of subtle fluctuation of the margins of this abnormality in the setting of such inflow artifact. In patients with a mild allergy to iodinated contrast, a corticosteroid premedication regimen should be administered. A history of anaphylactic reaction to iodinated contrast is often considered an absolute contraindication. Adequate renal function is necessary, unless the patient has end-stage renal disease [1]. Conventional venography uses ionizing radiation to generate the images, and the dose can vary widely based on the indication.
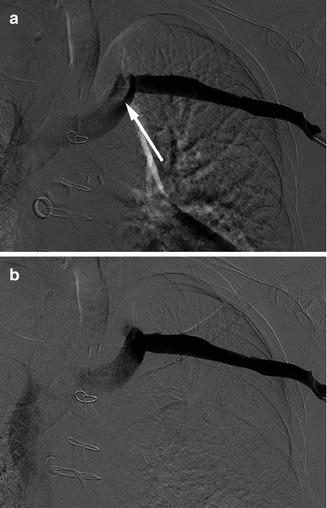
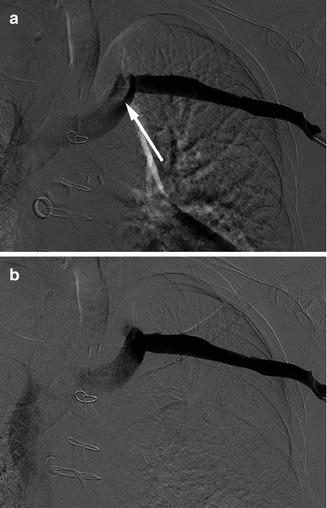
Fig. 9.3
Inflow artifact on a left upper extremity conventional venogram. (a) In this subtraction image, there appears to be a filling defect (arrow) in the left brachiocephalic vein. (b) However, a subsequent frame from this set of images reveals absence of a filling defect, which was caused by inflow of non-opacified blood via the left internal jugular vein
In summary, while conventional venography is considered the gold standard imaging modality for venous imaging, it is not typically utilized as first-line imaging for evaluating venous pathology. Conventional venography is often used in cases where endovascular therapy is anticipated, such as acute venous thrombosis (for thrombolysis) or venous stenosis requiring angioplasty or stenting. If other modalities have failed to diagnose an underlying venous process with certainty, then conventional venography can be used to obtain a definitive diagnosis. Some centers still utilize conventional venography as first-line imaging for upper extremity venous mapping for surgical hemodialysis access planning. If the patient has tenuous renal function or anaphylaxis to iodinated contrast, then special measures or alternative imaging modalities should be considered.
9.3 Computed Tomographic Venography
Computed tomography (CT) is well established as a method for three-dimensional imaging of the human body. CT images are generated based on the varying densities of different tissues. CT angiography (CTA), although well established as an excellent modality for imaging the arterial system, can also be used specifically for imaging the venous system (CT venography [CTV]). Both direct imaging and indirect imaging techniques can be used. Direct imaging entails injecting iodinated contrast in the venous distribution of interest and acquiring images as the contrast flows from the IV to the right atrium (Fig. 9.4). Indirect imaging entails injection of contrast into any vein (or central venous catheter), waiting several minutes to allow the contrast to reach the heart, recirculate through the arterial system, and back into the venous system [5]. Image acquisition is then performed as the contrast has opacified the venous system in its second pass (Fig. 9.5). By doing so, all veins in the body may potentially be imaged with a single injection, but at the expense of marked dilution of the injected contrast, which may limit or prohibit visualization of the vein(s) of interest. CTV image acquisition is the fastest of any modality, but it is performed with a set protocol with little flexibility.
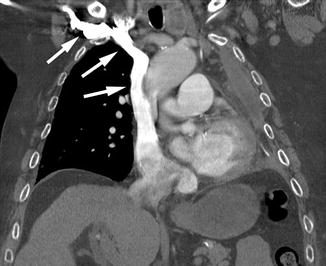
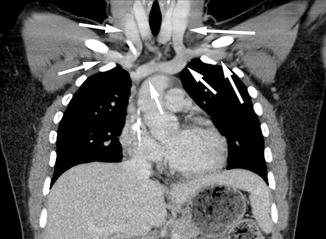
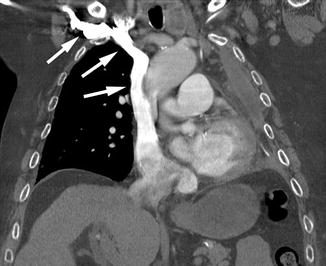
Fig. 9.4
Direct CTV image from a right arm venous injection. Note the extremely dense contrast in the right-sided central veins (arrows), but without significant opacification of any other veins
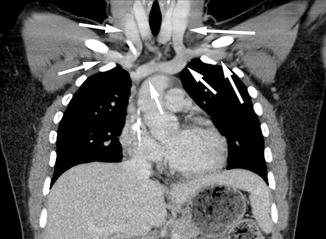
Fig. 9.5
Indirect CTV image from a right arm venous injection. The opacification of the right-sided central veins is much less dense, but all veins in this image are opacified (arrows)
Currently, multidetector CT scanners are typically used, which allows rapid scanning of areas of interest with minimal motion artifact due to breathing, etc. Although an 18–20 gage IV is ideal for automated power injection of contrast, a smaller IV with hand injection may be adequate for many cases. For imaging of the thoracic central veins, the patient needs to be able to hold their breath for a short period of time. As with conventional venography, appropriate renal function is required and a history of potential allergy should be elicited. A large body habitus can limit imaging quality due to attenuation of the radiation beam. Metallic implants such as prosthetic joints cause substantial “streak artifact” which can obscure visualization of the nearby venous structures. Appropriate protocols should be developed to obtain the greatest contrast opacification of the desired venous system.
The largest body of literature supporting CTV is for the detection of lower extremity DVT, often performed concurrently with pulmonary arterial imaging [6]. With this form of imaging, intravenous contrast is injected into an upper extremity IV, and pulmonary arterial imaging is first obtained to detect the presence of pulmonary emboli. A few minutes after contrast injection, the abdomen, pelvis, and lower extremities are then scanned for the presence of DVT (indirect imaging) [6, 7]. With this technique, CTV of the lower extremities has been shown to have a very high sensitivity and specificity for detection of DVT. However, due to the radiation dose and questionable incremental yield when the CTA reveals pulmonary embolism, this dual CTA/CTV protocol is no longer commonly used. The classic imaging finding for DVT is an occlusive filling defect which causes expansion of the vein (Fig. 9.6). In areas where a significant amount of fat surrounds the vein, acute DVT often causes increased density of the fat, termed “fat stranding.” Peripheral to the DVT, lower extremity edema is often present, as manifested by diffuse fat stranding, skin thickening, and thickening of subcutaneous septa. However, lower extremity edema is a nonspecific finding which can be caused by numerous other causes, such as volume overload, hypoalbuminemia, and others. Varicose veins can also be fairly well visualized, and thus, CTV has been reported to have significant utility for preoperative planning [8, 9]. CTV can also be helpful for evaluating central venous pathology. Occlusions and stenosis of the central veins can be well-depicted, as are any associated obstructive masses.
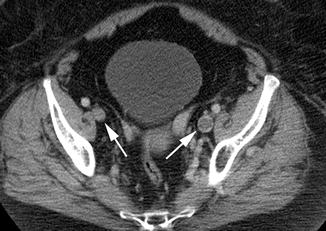
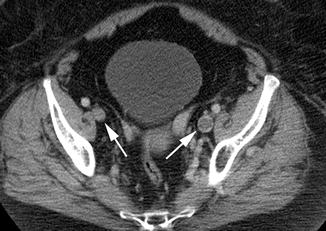
Fig. 9.6
CTV image showing an acute DVT in the left external iliac vein. This manifests as a filling defect (less density than expected due to the absence of intravenous contrast). Additionally, the clot is expansile, resulting in a larger diameter compared to the contralateral external iliac vein. Arrows denote the bilateral external iliac veins
Advantages of CTV include three-dimensional reconstruction and multiprojectional reconstruction which allow excellent evaluation of venous pathology and the presence of collateral veins [9]. Using specialized software, structures not of interest (bones and organs) can be removed from the image (Fig. 9.7). Any structural abnormalities impacting the venous system are well evaluated with CT, allowing diagnosis of masses and normal variant anomalies. Additionally, nonvascular pathology may be revealed, providing an alternate or additional diagnosis. Using indirect imaging, the entire bilateral venous system can be evaluated with a single contrast bolus under ideal conditions.
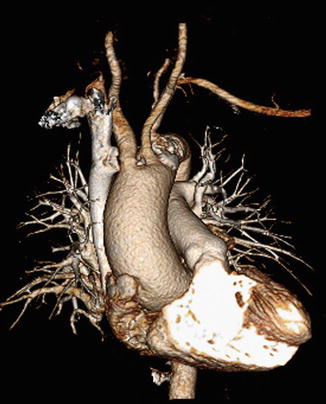
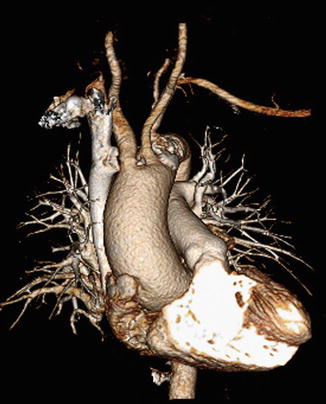
Fig. 9.7
Three-dimensional processed CTA image
The primary disadvantage of indirect CTV is the low level of venous opacification, which may not be adequate for diagnosing certain types of venous pathology, particularly intraluminal pathology. To the contrary, while direct CTV provides excellent venous opacification in the injected venous pathway, inflow artifact predisposes this method to false-positive studies. Additionally, with direct CTV of an upper extremity, the contralateral upper extremity veins and lower extremity veins will not be visualized. While utilization of both direct and indirect CTV in the same study will help alleviate these disadvantages, a double dose of radiation is required. Although the ionizing radiation dose to the lower extremities is generally of little concern in terms of carcinogenesis, the organs in the thorax and abdomen are more radiation sensitive, and thus radiation dose becomes of more significant concern for imaging these areas. And finally, the concentration of contrast in the vein may be excessive to the point that streak artifact occurs with associated image degradation (Fig. 9.8).
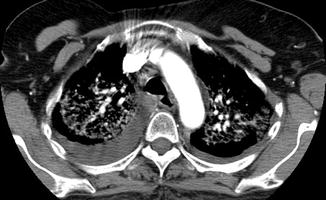
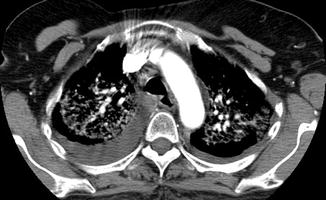
Fig. 9.8
Streak artifact caused by a high concentration of contrast in the superior vena cava. This star-like artifact obscures visualization of the adjacent structures
CTV can, in experienced centers, provide valuable diagnostic information of the venous system for a variety of indications. Because of the limitations as discussed above, CTV is not typically a first-line imaging modality. However, in cases where surrounding structures are important to the diagnosis, and when high spatial resolution is crucial, CTV can provide excellent diagnostic information without need for other adjunct studies.
9.4 Magnetic Resonance Venography
Magnetic resonance imaging (MRI) combines an extremely strong magnet to align the polar molecules in the body with repetitive radiofrequency pulses to alter the alignment. The resulting differences in electromagnetic signal are then processed into an image. Numerous technical parameters can be adjusted to optimize visualization of any type of tissue in the body. For visualization of blood, both contrast-enhanced techniques as well as non-contrast techniques can allow excellent visualization of vessels (Fig. 9.9). When contrast is used, gadolinium-based agents are by far most common.
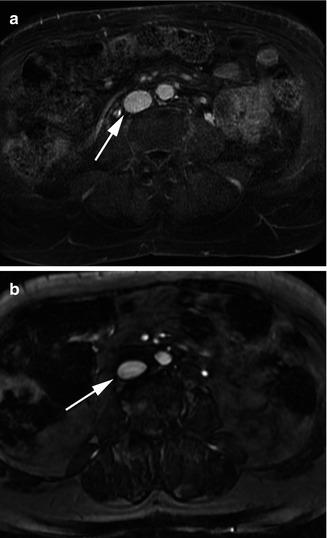
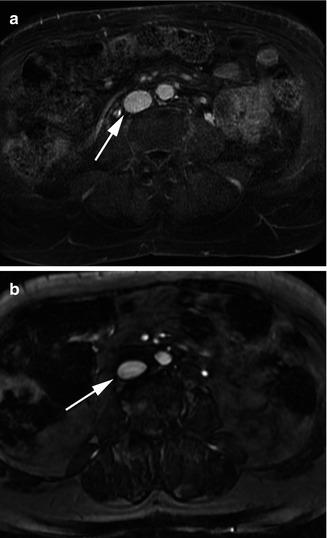
Fig. 9.9
MRV through the inferior vena cava (arrow). (a) Contrast enhanced. (b) Non-contrast
The physics involved in MRI are complex and multiple textbooks have been dedicated to explain the technology behind it. An extremely strong magnet is the basis of this imaging modality, typically in the range of 1.5–3 Tesla (T) strengths. Dedicated coils, specialized apparatus placed upon the body, are used to maximize signal detection for the various body parts. During imaging, patients need to lie motionless in the supine position within the magnet, or else the image quality will be significantly degraded. For imaging of the chest and abdomen, intermittent breath-holding is necessary. Although numerous gadolinium-based agents can be used for MRV, the use of a blood-pool agent may be best suited for venous imaging (Fig. 9.10). This agent, by virtue of albumin binding, is retained in the intravascular space markedly longer than regular gadolinium agents. By this principle, the contrast is more highly concentrated in veins, which allows superior venous imaging quality. Furthermore, due to its long intravascular retention time, timing of image acquisition in relation to the time of contrast injection is no longer a difficult task, and prolonged imaging can be performed which can markedly improve spatial resolution. Another helpful technique for venous imaging is time-resolved MRA, which is characterized by the rapid acquisition of three-dimensional image datasets at three- to five-second time intervals over the course of several minutes after the injection of contrast. By doing so, the images can be viewed in a real-time manner, which allows visualization of the flow dynamics, similar to conventional venography (Fig. 9.11). This can provide valuable physiologic information of the venous system. Numerous non-contrast MRA techniques are available, which work via varying mechanisms, and thus have varying advantages and disadvantages. Currently, the most popular non-contrast techniques include “time-of-flight” and “steady-state free precession” pulse sequences, which result in high signal in the blood vessels. Due to the risk of nephrogenic systemic fibrosis, discussed in further detail later in the chapter, an appropriate glomerular filtration rate is needed if gadolinium-based contrast is going to be used. According to the Federal Drug Administration guidelines, an estimated glomerular filtration rate of less than 30 mL/min/1.73 m3 is a contraindication to administration of gadolinium contrast.
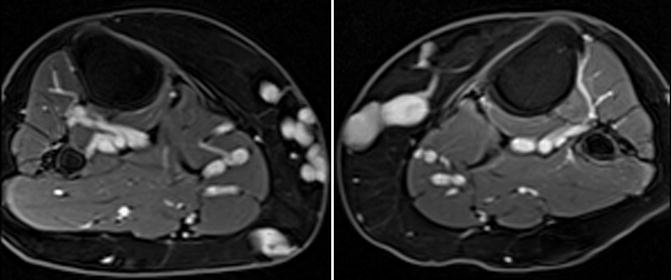
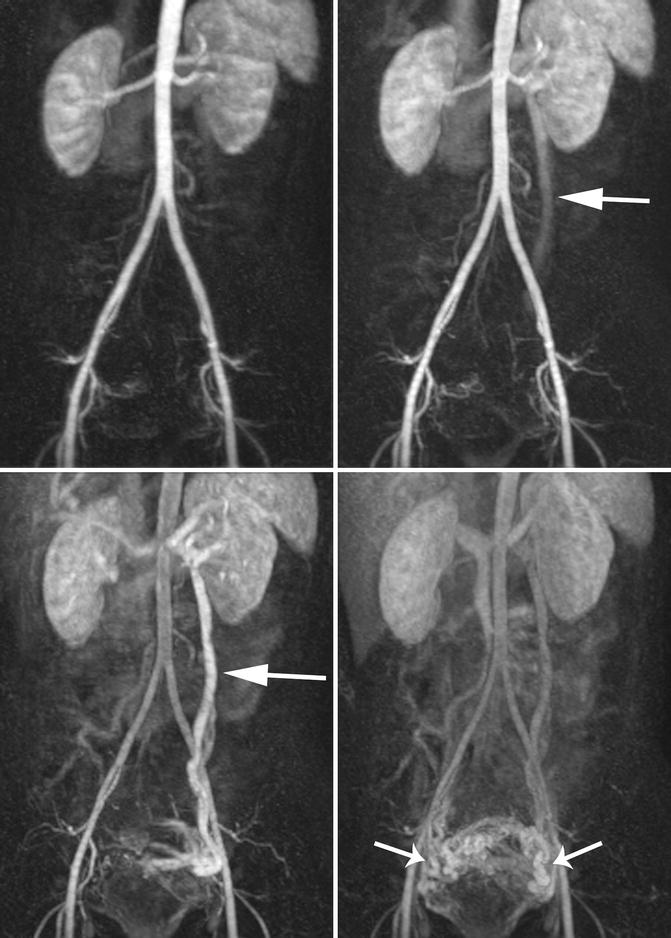
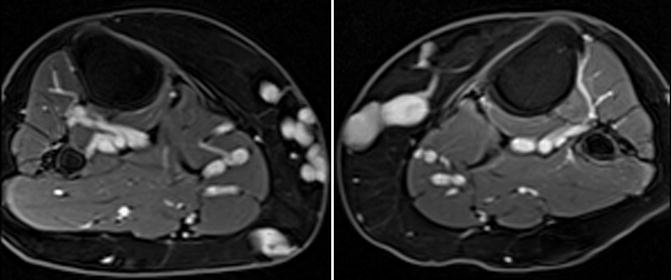
Fig. 9.10
Contrast-enhanced MRV of the thighs with a blood-pool agent allows excellent venous visualization with high detail
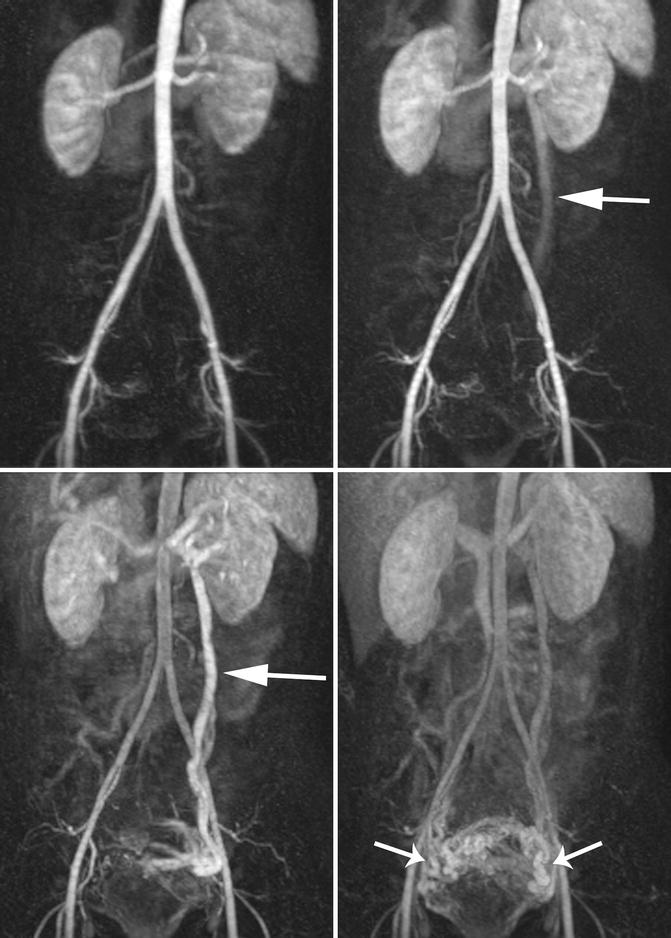
Fig. 9.11
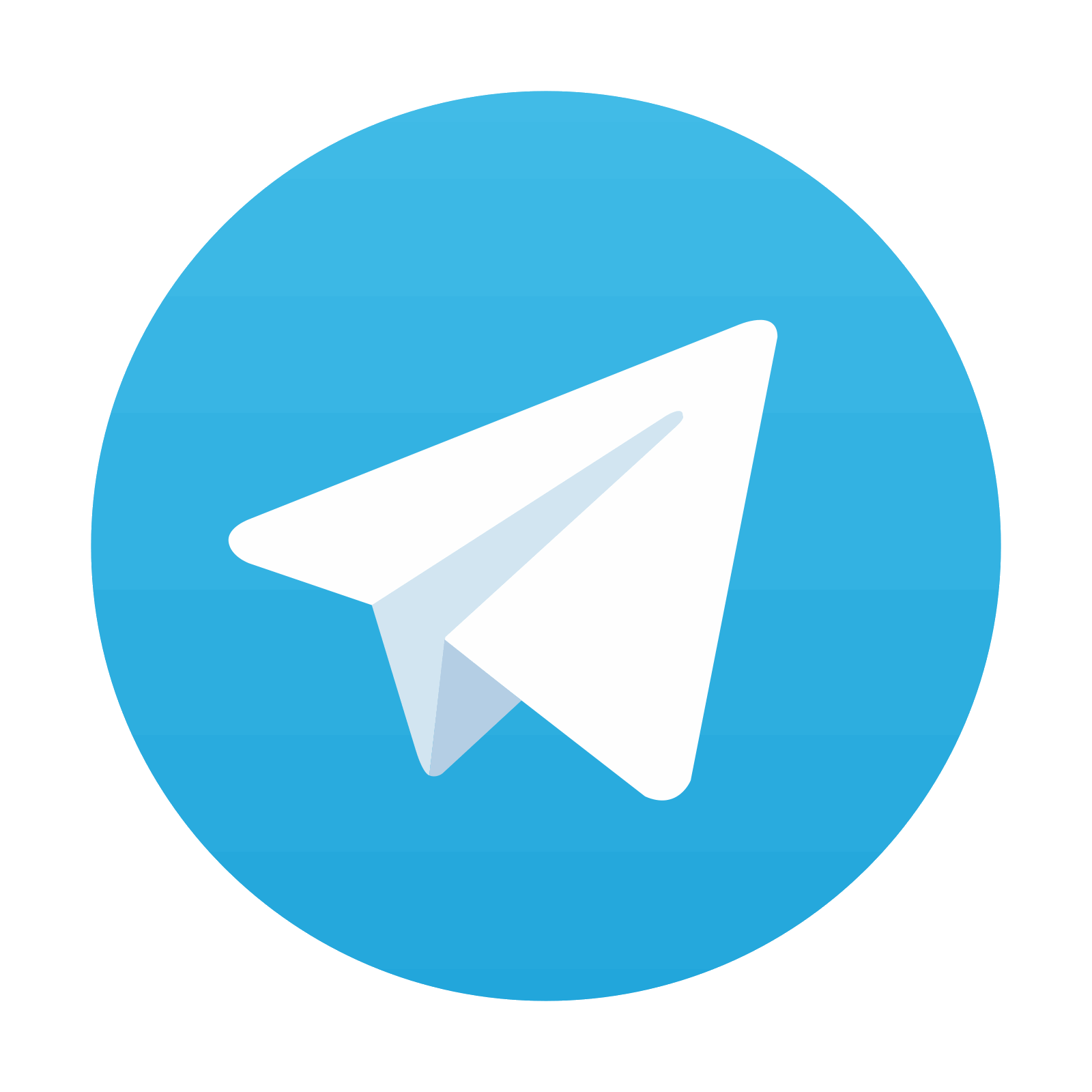
Time-resolved MRV of the abdomen and pelvis allows visualization of the blood flow dynamics. (a) Arterial phase image. (b, c) Venous phase images demonstrate progressive opacification of the left ovarian vein (arrow) towards the ovaries, which is in the wrong direction. (d) Late venous phase images demonstrate opacification of periuterine venous varices (arrows). This constellation of findings is compatible with pelvic congestion syndrome
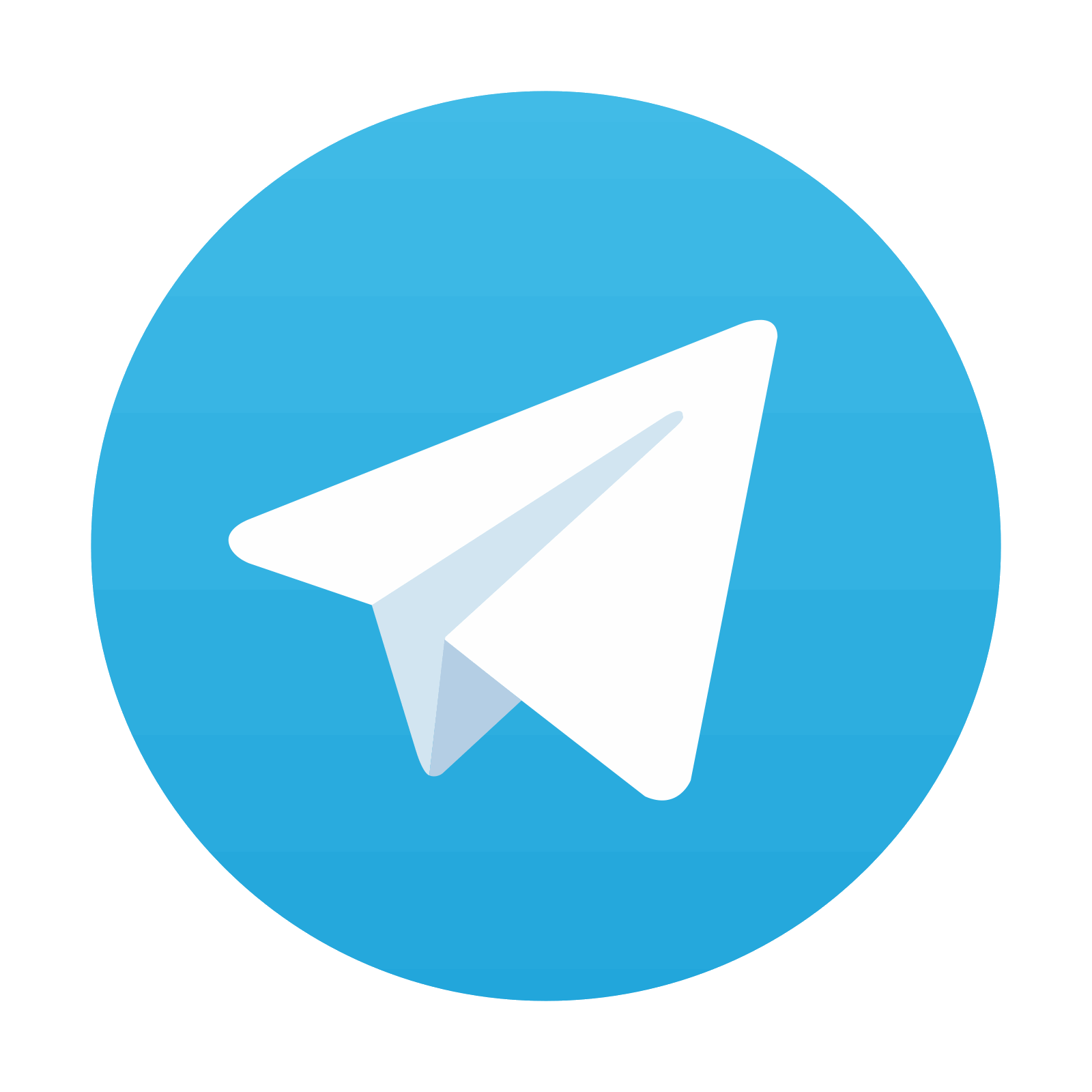
Stay updated, free articles. Join our Telegram channel
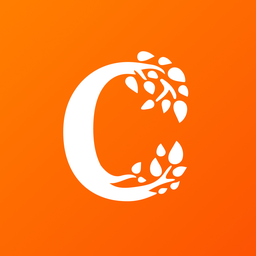
Full access? Get Clinical Tree
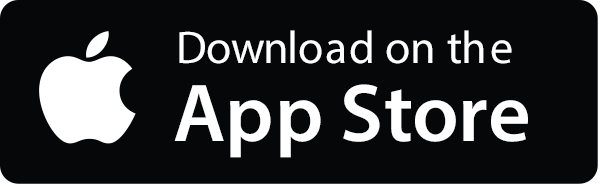
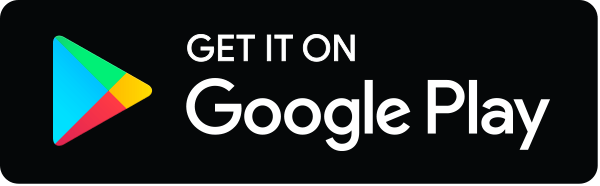
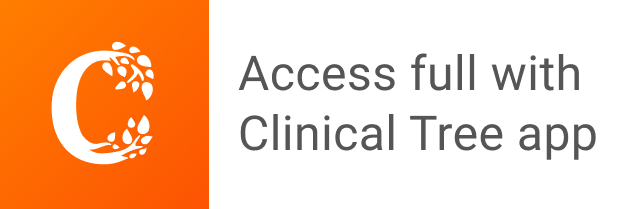