Control of Ventilation
INTRODUCTION
Breathing is a rhythmic motor act, which is under both conscious and automatic control. This system maintains numerous controlled variables within their homeostatic ranges, but is also responsible for rapidly changing ventilation in response to often unpredictable stimuli. We will discuss the anatomy and physiology of the ventilatory control system, then address integrated responses and illustrative examples of adaptation and dysfunction in the setting of selected disease states.
ANATOMY AND PHYSIOLOGY
The respiratory control system, broadly speaking, comprises a controller, sensors, and a plant (Fig. 11-1). This hierarchical structure, in which there is central processing of afferent input, is important for coordinating respiratory movements with behaviors such as eating, speaking, and moving.1 The controller is a neuronal network within the central nervous system (CNS), which is responsible for generating and modulating individual breaths and the overall breathing pattern. Often referred to as the respiratory central pattern generator (rCPG), the controller comprises reciprocally connected neuronal populations in the medulla and pons.2,3 Neural output from the rCPG drives the activity of various motor neuron pools. Motor neurons in the spinal cord (e.g., phrenic and intercostal) innervate the respiratory pump muscles, while brain stem motor neurons innervate upper airway muscles. The so-called “plant” includes the CO2 stores, which are made up of lung stores and circulating blood volume including hemoglobin, and is an important component of breathing control. Closed loop feedback to the controller is supplied by chemoreceptors and mechanoreceptors.
Figure 11-1 Block diagram of the respiratory control system.
The consistent cycling of the ventilatory pattern is generated spontaneously from the spatial and functional architecture of the rCPG. Intrinsic membrane properties of rhythmically active neurons within the rCPG are capable of producing automatic periodicity.4 In addition, reciprocal (excitatory and inhibitory) synaptic connections between neuronal populations in the medulla and pons are believed to be critical for the automatic generation of the respiratory rhythm.2,3
The neural respiratory cycle comprises three phases (Fig. 11-2).5 Inspiration (TI) involves ramp-like increases in inspiratory motor neuron firing, which drive phrenic nerve activity throughout this phase. The first phase of expiration (TE1) is often called post-inspiration, because inspiratory motor neurons are still active. Persistent inspiratory motor activity during TE1, which declines throughout this phase, acts to slow the exit of air from the lungs. Finally, during the second phase of expiration (TE2), expiratory muscles are typically electrically silent. During this phase of passive relaxation, gas is expelled as the lungs and chest wall return to their equilibrium state (i.e., functional residual capacity). However, under conditions where respiratory drive is increased, expiratory muscles including the internal intercostal and abdominal muscles become active during TE2. This notion is an example of how the central controller, influenced by sensory feedback, modulates and alters the integrated motor response of the system.
Figure 11-2 Recording of phrenic nerve activity (below) and its moving average (above), which highlights the three phases of the respiratory cycle: (1) Inspiration (I), (2) post-inspiratory activity (expiration, phase 1, E1), and (3) late expiration (expiration, phase 2, E2). PIIA, post-inspiratory inspiratory activity.
BRAIN STEM
Within the medulla and pons, interconnected neuronal populations constitute a network, which is considered necessary for the generation of the respiratory rhythm (Fig. 11-3).2,3,6 This bilateral pontomedullary respiratory network, which is also responsible for control of the ventilatory pattern, contains the ventral respiratory column (VRC) and the pontine respiratory group (PRG). Afferent signals from lung mechanoreceptors and peripheral chemoreceptors enter the pontomedullary network via the nucleus of the solitary tract (nTS) in the dorsal respiratory group (DRG). The nTS has projections to both pontine and ventral medullary components of the central respiratory network. Taken together, the VRC, DRG, and PRG comprise the brain stem rCPG. The rhythmic output of the rCPG drives the activity of spinal phrenic, intercostal, and lumbar motor neuron pools that innervate the muscles of respiration. Other brain stem motor neurons exhibit respiratory modulation and control muscles of the upper airway. Finally, the rCPG is influenced by higher CNS structures, which allows for conscious control of the ventilatory pattern.
Figure 11-3 Overview of the respiratory central pattern generator (rCPG) within the brainstem. The ventral respiratory column (VRC) is orientated in the rostral-to-caudal direction. The VRC extends from the retrotrapezoid nucleus (RTN) adjacent to the rostral facial nucleus (VII) superiorly, to the caudal ventral respiratory group (cVRG) near the spinomedullary junction inferiorly. The main areas of rhythmically active VRC respiratory neurons are in the Bötzinger complex (BötC), pre-Bötzinger complex (pre-BötC), rostral ventral respiratory group (rVRG), and cVRG. The parafacial respiratory group (pFRG) overlaps anatomically with the RTN, and together these regions include intrinsically bursting neurons which may contribute to rhythm generation. Peripheral chemo- and mechanosensory inputs are transmitted to the nucleus of the solitary tract (nTS) in the dorsal respiratory group (DRG). The pontine respiratory group (PRG) includes the Kölliker–Fuse (K–F) and the parabrachial (PB) nuclei which contain respiratory-modulated neurons. See text for additional details.
Several regions of rhythmically active neurons in the VRC represent the core circuitry of the rCPG. The pre-Bötzinger complex (pre-BötC) within the VRC is believed to be the main source of rhythmic excitation driving inspiratory premotor neurons and other brain stem circuits. Furthermore, the pre-BötC has been shown to manifest intrinsic rhythmic activity.4 However, the mechanisms responsible for rhythmic inspiratory pattern generation are complex given the large number of modulatory inputs that converge on the pre-BötC compartment, and are likely to manifest as the result of network properties. Neuronal populations in the Bötzinger complex (BötC) are the major source of expiratory network activity during normal breathing. Thus, BötC neurons are important for control of the transition between inspiratory and expiratory activities in the rCPG and maintenance of the rhythmicity of normal breathing.
Neurons within the PRG are critical for formation of normal resting breathing patterns. Functional connections between the pons and the rest of the rCPG modulate phase switching (onset and termination of inspiration). For example, the dorsolateral pons (dlPons) is a region within the PRG with respiratory-modulated neurons whose activity varies depending on the presence of vagal afferent feedback. In particular, eupneic breathing patterns are dependent on excitatory drive from pontine neurons to the VRC, emphasizing the importance of the spatial and functional architecture of the brainstem respiratory network. Medullary raphé nuclei likely represent a system of intermediate relays for signaling between the PRG and the VRC. Transmission of an efference copy of ventilatory drive from the VRC is an example of one of these reciprocal connections. These medullary circuits may serve to maintain an overall level of rCPG activity. In addition, certain (particularly rostral) regions within the medullary raphé are chemosensitive, responding to local changes in carbon dioxide or pH, as well as exhibiting altered activity with stimulation of peripheral chemoreceptors.
The rostral ventral respiratory group (rVRG) region within the VRC comprises excitatory neurons that drive spinal phrenic and intercostal inspiratory motor neurons. This group of neurons is inhibited by the BötC during expiration and excited by the pre-BötC during inspiration. These rhythmically alternating influences, along with modulatory inputs from other areas of the pontomedullary network, are responsible for shaping and controlling the pattern of inspiratory rVRG activity. In contrast, the cVRG is thought to be the expiratory counterpart to rVRG activity.
Peripheral chemo- and mechanosensory inputs are transmitted to the nTS, which contains second-order neurons critical for reflex respiratory responses. For example, carotid body chemoreceptor and baroreceptor afferents terminate in the medial and lateral subnuclei of the nTS. Lung mechanoreceptors have projections to “pump cells” in the nTS, resulting in rhythmic activity of these cells which is modulated by lung inflation. The retrotrapezoid nucleus (RTN) is a site of central chemoreception. Chemoresponsive neurons within this region project to other areas of the rCPG and provide excitatory drive to the VRC and PRG. These sensory afferents, and the way in which they modulate breathing, will be discussed in subsequent sections. As part of the respiratory neural network, the nTS modulates breathing via projections to both pontine and ventral medullary components of the central respiratory network. In addition, neurons within the nTS receive inspiratory drive from the VRC and reciprocal pontine projections gate neuronal activity in the nTS.
CHEMORECEPTORS
The carotid and aortic bodies are bilateral sensory organs that detect changes in arterial oxygen. Although very small in size, the reflexes initiated by these tissues (particularly the carotid bodies) are critical for evoking stimulation of breathing during hypoxemia.7 Stimulation of the carotid body by hypoxia (O2 sensing) involves stimulus transduction and afferent nerve activation. Carotid bodies are composed of two cell types: type I and type II. Type I cells (also referred to as glomus cells) are of neural crest origin and are considered the putative oxygen-sensing cells. Glomus cells express a variety of neurotransmitters that play critical roles in sensory transmission of hypoxemia. Afferent nerve endings, whose cell bodies lie in the petrosal ganglion, form synaptic contacts with glomus cells and are responsible for afferent signaling to the CNS. The type II cells (also referred to as sustentacular cells) resemble glial cells and they are thought to act primarily as supporting cells.
There are two main pathways by which the carotid body senses hypoxia, although which mechanism is primary remains an area of debate.8–10 The first main pathway for oxygen sensing involves heme-containing proteins in the glomus cell (metabolic hypothesis). First proposed by Mills and Jobsis, and later supported by studies using exogenous carbon monoxide (CO), there is evidence that mitochondrial cytochrome(s) may act as potential O2 sensors. Further, several nonmitochondrial heme proteins that are expressed in glomus cells, including NADPH oxidases and heme oxygenase-2 (HO-2), have been proposed as potential oxygen sensors. Finally it has been suggested that hypoxia leads to the formation of iron-containing compounds via chelation as part of the transduction process, although the putative proteins and downstream signaling pathways responsible for afferent nerve activation are not well defined.
The second general mechanism by which hypoxia leads to depolarization of glomus cells is via inhibition of an O2-sensitive, membrane-bound K+ channel. This pathway for sensory transduction is based on the neuronal phenotype of the glomus cells and is referred to as the membrane hypothesis. Glomus cells express a variety of O2-sensitive K+ channels including outward rectifiers, Ca2+-activated K+ channels, human-ether-a-go-go (hERG), and twin pore-acid–sensitive K+ (TASK) channels.11 There is published evidence supporting and refuting aspects of both the metabolic and membrane hypotheses. Most likely, both pathways complement each other and work in concert to facilitate oxygen sensing by glomus cells. For example, it has been proposed that with increasing severity of hypoxemia, additional pathways are activated, allowing the carotid body to respond to a wide range of arterial oxygen levels.
Regardless of the mechanism(s) involved, hypoxic sensing ultimately leads to a Ca2+-dependent release of neurotransmitters from glomus cells. These signaling molecules activate nerve endings in the carotid sinus nerve leading to an increase in afferent nerve activity (sensory transmission). The carotid body expresses several classes of neurotransmitters, including (1) biogenic amines (acetylcholine [Ach], dopamine, norepinephrine, and 5-hydroxytryptamine), (2) neuropeptides (enkephalins, substance P, and endothelins), (3) adenosine triphosphate (ATP), (4) amino acids (e.g., GABA), and (5) gas transmitters (CO and nitric oxide, NO). Some of the transmitters (e.g., ACh, substance P, ATP) stimulate whereas others (e.g., dopamine, enkephalins) inhibit carotid sinus nerve activity. Identifying which transmitters are primarily responsible for hypoxia-induced afferent nerve activation under physiological conditions remains an area of active research, and it is possible that corelease of multiple molecules, acting in concert, may ultimately be responsible for sensory excitation by hypoxia. While the carotid body is the primary peripheral chemoreceptor responsible for oxygen sensing, carbon dioxide also stimulates the carotid body, but the mechanisms are not fully understood. Finally, the interaction of the central and peripheral chemoreceptors is still debated, but recent evidence supports a hyperadditive model whereby the gain of one group of chemoreceptors can increase the responsiveness of the other (e.g., carotid body stimulation increases the gain of the central chemoreceptors).12
MECHANORECEPTORS
The lung, chest wall, and respiratory muscles all contain mechanoreceptors, which provide closed-loop feedback to the rCPG. This afferent input regulates minute ventilation independent from chemical drive. In addition, mechanoreceptor reflexes modulate the respiratory pattern on a breath-by-breath basis by enhancing or terminating inspiration. Afferent axons from lung mechanoreceptors are contained within the vagus nerve, but the importance of these receptors to control of respiration in awake, adult humans is likely small. Additional mechanoreceptors in the respiratory muscles and chest wall have central projections which travel in spinal nerves and the spinal cord. These receptors are important to coordinating the breathing pattern during trunk twisting and during movement between the upright and supine positions.
Several broad categories of mechanoreceptors have been identified which are relevant to ventilatory control:
1. Lung stretch receptors are present in the airway smooth muscle of the distal airways. These slowly adapting receptors are stimulated by lung inflation. Afferent signaling following activation of these receptors tends to terminate inspiration without altering the slope of the inspiratory ramp, as well as to promote expiratory activity. Slowly adapting receptors in the lung are the afferent fibers responsible for the Hering–Breuer reflex, which describes the termination of inspiration (promotion of inspiratory-to-expiratory phase switching) that occurs as a result of lung inflation. In addition, expiratory prolongation and occasional apneas which occur with large and sustained inflation of the lung are a manifestation of this reflex.
2. Rapidly adapting receptors respond to changes in airway mechanical properties that accompany lung inflation and deflation, and become more active as the rate of airflow increases. These receptors are also activated by stimuli that induce bronchospasm, edema, or mucus secretion. For example, they produce cough and laryngeal narrowing in response to stimulation by dust, ammonia, histamine, and other agents, as well as in response to increases in inspiratory airflow. These lung mechanoreceptors are primarily located in the epithelial and submucosal layers of the larger airways, accounting for their sensitivity to inhaled agents. Finally, rapidly adapting receptors lead to increased respiratory frequencies at low lung volumes, due to afferent signaling which increases inspiratory neural activity during lung deflation.
3. Bronchial J receptors are named for their juxtacapillary location. These lung mechanoreceptors project centrally through unmyelinated (in contrast to the first two types) fibers, and respond to pulmonary vascular congestion associated with increases in pulmonary artery and capillary pressure. Stimulation contributes to the increase in respiratory frequency and a decrease in tidal volume in response to pulmonary edema.
4. Bronchial C receptors are named for their sensitivity to capsaicin, and are located in the airway wall. Similar to type J receptors, these lung receptors project centrally through unmyelinated fibers. Activation of C fibers with capsaicin or bradykinin produces cough and a rapid, shallow breathing pattern. Unlike other lung receptors described here, bronchial C receptors are relatively insensitive to mechanical stimulation and changes in lung volume.
5. Muscle spindles transduce muscle length. The diaphragm has few if any muscle spindles, but these mechanoreceptors are plentiful in the intercostal muscles. Muscle spindles tend to augment breathing when activated.
6. Muscle tendon organs are located in series with muscle fibers in the tendon and are activated as muscle fibers generate force. Thus, these mechanoreceptors sense the efficiency of force generation and inhibit breathing when activated. The central tendon of the diaphragm contains tendon organs, as do the tendons of accessory muscles of respiration.
7. The rib cage joints and upper airways (larynx, pharynx, and nasal cavity) also contain receptors that impact control of ventilation. For example, cold air stimulation of the pharynx initiates the cough reflex, and can reduce the ventilatory response to carbon dioxide.
UPPER AIRWAY AND PUMP MUSCLES
The upper airway muscles are described more completely elsewhere but are addressed briefly here for completeness. The pharyngeal dilator muscles can be broadly classified into those with phasic activity (burst with inspiration) and tonic activity (constant activity throughout the respiratory cycle). The genioglossus is a frequently studied muscle as it is a representative phasic muscle which is readily accessible for intramuscular recordings and is controlled by the hypoglossal motor nucleus. The genioglossus is a major dilator muscle, which serves to protrude the tongue and protect pharyngeal patency in the face of collapsing perturbations. The tensor palatini, in contrast, is a representative tonic muscle controlled by trigeminal motor branches. Recent insights from recordings of single motor units have defined the complexity of these various muscles and provided insights into brainstem control in humans.13 Although robust activity of the pharyngeal dilator muscles have been observed in obstructive sleep apnea patients during wakefulness, the fall in activity of these muscles at sleep onset likely contributes to a propensity to upper airway collapse in susceptible individuals. Some data also support evidence of structural neural remodeling and reinnervation of genioglossus muscle fibers suggesting neural injury may also be present in people with sleep apnea.14 Given the recent realization that sleep apnea has multiple underlying mechanisms, there is likely a subset of OSA patients who have dysfunction in upper airway muscles as a major pathogenic factor. Various approaches are being considered to augment upper airway dilator muscle activity, including the possibility of pharmacological manipulation of hypoglossal output or electrical stimulation of the hypoglossal nerve. Pharmacological targets are being defined through careful studies of premotor inputs to the hypoglossal motor nucleus, although as yet no human trials have shown clear augmentation of genioglossus activity with pharmacotherapy. While the role of hypoglossal nerve stimulation in treating sleep apnea remains incompletely defined, recent work highlights the clinical promise of this approach.15
STABILITY OF VENTILATORY CONTROL: LOOP GAIN
Loop gain is an engineering term that is used to define the stability or instability of a negative feedback control system. Overall loop gain can be thought of as the integration of controller and plant factors and is thus the product of controller gain and plant gain (Fig. 11-1). A system with a high loop gain is prone to instability whereas a system with low loop gain is one which is intrinsically stable. The concept of loop gain can be considered in the context of the thermostat analogy, which is a common example of a negative feedback control system designed to regulate room temperature. Situations that lead to oscillations in room temperature can be considered analogous to situations in humans in which CO2 levels fluctuate. For example, a highly sensitive thermostat is one that responds to trivial fluctuations in room temperature with major changes in output from the air conditioner or furnace; thus, a very sensitive thermostat will lead to marked fluctuations in room temperature. By analogy, exquisite sensitivity of chemoreceptors will contribute to marked fluctuations in CO2 levels. Another example is a furnace, which is too powerful such that a minor drop in room temperature leads to major elevations in room temperature as a result of the output of the furnace. By analogy, if a minor increase in PaCO2 led to major increases in ventilation yielding marked reductions in PaCO2, the system would be considered unstable, that is, elevated loop gain. Thus, situations, which lead to fluctuations in room temperature, can be considered to understand the factors underlying CO2 fluctuations in the human.
In the case of ventilation, the propensity for CO2
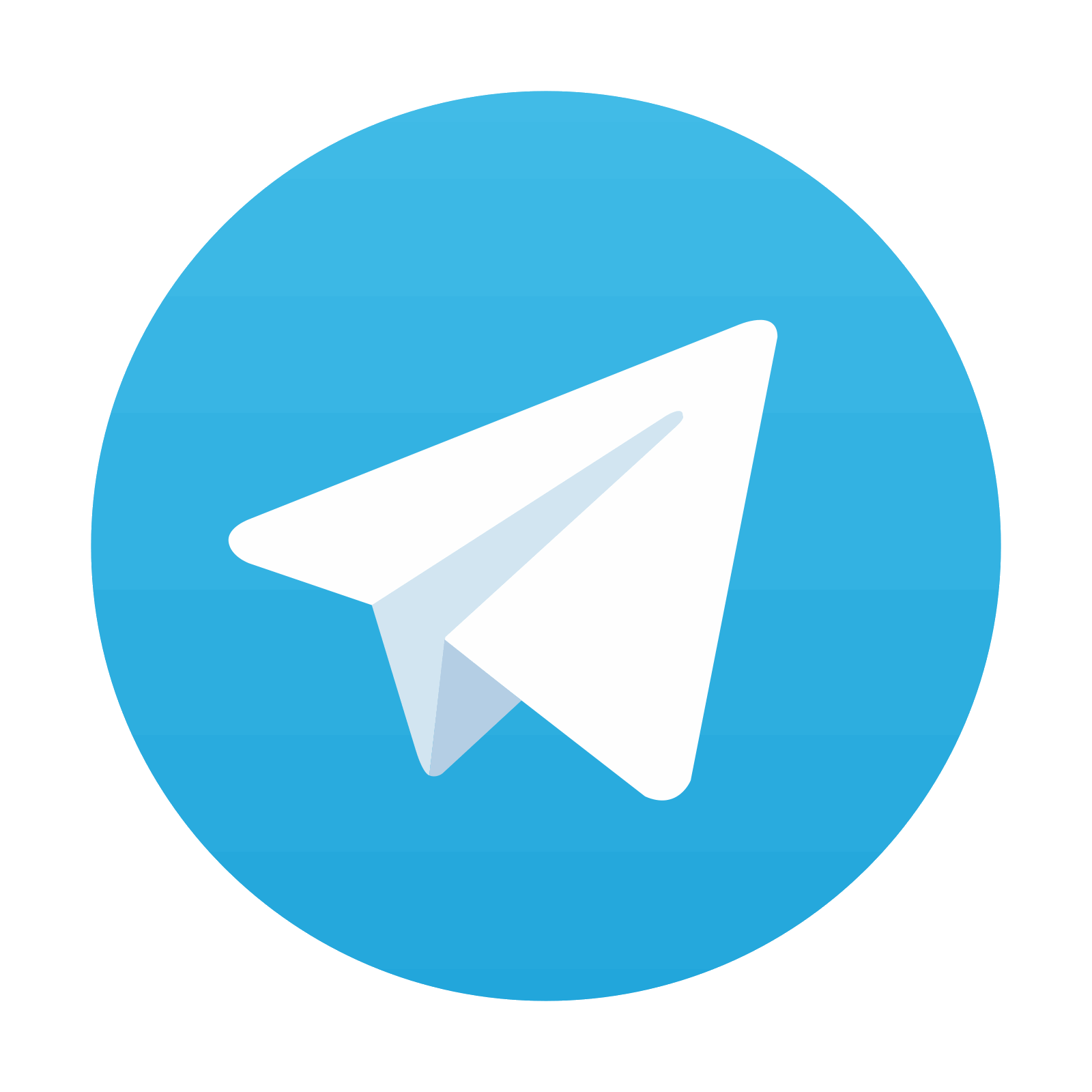
Stay updated, free articles. Join our Telegram channel
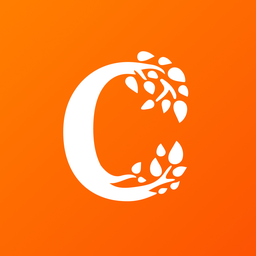
Full access? Get Clinical Tree
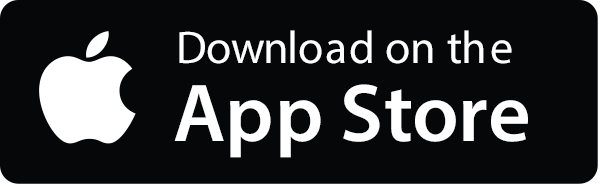
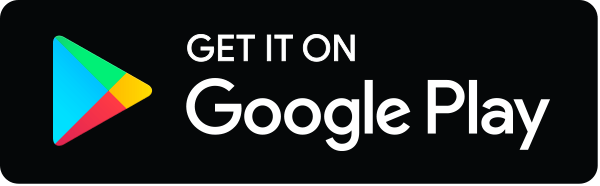