Location
Type of receptor
Effects of simulation
Heart
Sinus node
β1
Increase in heart rate
Atrioventricular node
β1
Increase in heart rate
Coronary circulation
α
Vasoconstriction
Coronary circulation
DA
Vasodilation
Peripheral vasculature
Pulmonary
α
Vasoconstriction
Renal
DA
Vasodilation
Mesenteric and splanchnic
DA, β1
Vasodilation
Skin
α
Vasoconstriction
Skeletal muscle
β1
Vasodilation
Nonvascular
Bronchial tree
β2
Bronchodilation
Renal tubule
DA
Diuresis
Table 41.1b
Physiological effects of different agents
Drug | Receptor | Effects | |||||||||
---|---|---|---|---|---|---|---|---|---|---|---|
α | β1 | β2 | DA | Na+/K+ ATPase | CO | Contractility | SVR | MAP | PCWP | HR | |
Dopamine | ++ | ++ | + | ++ | ↑↑ | ↑ | ↑↔↓ | ↑↔ | ↑↔ | ↑ | |
Dobutamine | + | +++ | + | ↑↑ | ↑↔ | ↓ | ↓↔ | ↔ | ↑↔ | ||
Isoproterenol | +++ | +++ | ↑↑ | ↔ | ↓↓ | ↓ | ↓ | ↑↑ | |||
Milrinone | ↑↑ | ↑ | ↓↓ | ↓↔ | ↑↔ | ↑↔ | |||||
Epinephrine | +++ | +++ | +++ | ↑↑ | ↑↔ | ↑↔↓ | ↑↔ | ↑↔ | ↔/↑/↑↑ | ||
Norepinephrine | +++ | + | ↑↔↓ | ↑ | ↑↑↑ | ↑↑ | ↑↔ | ↑/↑↑ | |||
Nitroprusside | ↑ | ↔ | ↓↓ | ↓ | ↓ | ↑↔ | |||||
Nitroglycerin | ↑ | ↔ | ↓ | ↓ | ↓↔ | ↑↔ | |||||
Enalapril | ↑ | ↔ | ↓ | ↓ | ↓↔ | ↑↔ | |||||
Digoxin | +++ | ↑ | ↑ |
Circulation
During cardiac arrest, chest compressions provide the sole perfusion to a child’s vital organs; therefore, optimal performance of CPR is critical. Key elements to providing quality chest compressions include (1) ensuring an adequate rate (100 compressions per minute), (2) ensuring adequate chest wall depression (one third to half of the anteroposterior chest diameter), (3) releasing completely between compressions to allow full chest wall recoil, (4) minimizing interruptions in chest compressions, and (5) ensuring that the child is on a sufficiently hard surface to allow effective chest compressions (ECC Committee, Subcommittees and Task Forces of the American Heart Association 2005). In short, push hard and push fast, release completely, and do not interrupt compressions unnecessarily. Incomplete recoil during CPR is associated with higher intrathoracic pressures and significantly decreased venous return, and coronary and cerebral perfusion (Berg et al. 2010).
If a child is small enough (e.g., younger than 6 months) that the person providing chest compressions can comfortably encircle the chest with his or her hands, chest compressions should be performed using the circumferential technique, with thumbs depressing the sternum and the fingers supporting the infant’s back and circumferentially squeezing the thorax. In larger infants, the sternum can be compressed using two fingers, and in the child, either one or two hands can be used, depending on the size of the child and of the rescuer (Berg et al. 2010). Whichever method is used, focused attention must remain on delivering effective compressions with minimal interruptions (Kleinman et al. 2010). In all cases other than circumferential CPR, a backboard must be used. Properly delivered chest compressions are tiring to the provider, and providers should rotate approximately every 2 min to prevent compressor fatigue and deterioration in the quality and rate of chest compressions (Berg et al. 2010).
Mechanisms of Blood Flow
External chest compressions provide cardiac output through two mechanisms: the cardiac pump mechanism and the thoracic pump mechanism. By the cardiac pump mechanism of blood flow, blood is squeezed from the heart by compression of the heart between the sternum and the vertebral column, exiting the heart only anterograde because of the closure of the atrioventricular valves. Between compressions, ventricular pressure decreases below atrial pressure, allowing the atrioventricular valves to open and the ventricles to fill. This sequence of events resembles the normal cardiac cycle. Although the cardiac pump is likely not the dominant blood flow mechanism during most closed-chest CPR, specific clinical situations have been identified in which the cardiac pump mechanism is more prominent. For example, a smaller, more compliant chest may allow for more direct cardiac compression. Increasing the applied force during chest compressions also increases the likelihood of direct cardiac compression.
Several observations do not support the cardiac pump as the primary mechanism of blood flow during CPR. Angiographic studies show that blood passes from the vena cava through the right heart into the pulmonary artery and from the pulmonary veins through the left heart into the aorta during a single chest compression (Niemann et al. 1981; Cohen et al. 1982). Echocardiographic studies show that the atrioventricular valves are open during blood ejection (Niemann et al. 1981; Werner et al. 1981; Rich et al. 1981). Without the closure of the atrioventricular valves during chest compression, the cardiac pump mechanisms cannot account for forward movement of blood during CPR.
In 1976, Criley and colleagues (1976) made the dramatic observation that several patients who developed ventricular fibrillation during cardiac catheterization produced enough blood flow to maintain consciousness by repetitive coughing (Criley et al. 1976). The production of blood flow by increasing thoracic pressure without direct cardiac compression describes the thoracic pump mechanism, in which the heart is a passive conduit for blood flow. The intrathoracic pressure is greater than the extrathoracic pressure during the compression phase of CPR, at which time blood flows out of the thorax, with venous valves preventing excessive retrograde blood flow. Experimental and clinical data support both mechanisms of blood flow during CPR in human infants.
Rate and Duty Cycle
The recommended rate of chest compressions for all patients is 100 per minute, with great care taken to minimize interruptions in chest compressions and to ensure adequate compression depth (Kleinman et al. 2010). This rate represents a compromise that attempts to maximize contributions from both the thoracic pump and cardiac pump mechanism of blood flow.
Duty cycle is defined as the percent of the compression–relaxation cycle that is devoted to compression. If blood flow is generated by direct cardiac compression, then primarily the force of compression determines the stroke volume. Prolonging the compression (increasing the duty cycle) beyond the time necessary for full ventricular ejection should have no additional effect on stroke volume. Increasing the rate of compressions should increase cardiac output, because a fixed volume of blood is ejected with each cardiac compression. In contrast, if blood flow is produced by the thoracic pump mechanism, the volume of blood that is ejected comes from a large reservoir of blood contained within the capacitance vessels in the chest. With the thoracic pump mechanism, flow is enhanced by increasing either the force of compression or the duty cycle but is not affected by changes in compression rate over a wide range of rates, given a set duty cycle (Chandra et al. 1990).
Different animal models yield conflicting results as to the optimal compression rate and duty cycle. However, a rate of compression during conventional CPR of 100 per minute satisfies both those who prefer the faster rates and those who support a longer duty cycle. This is true because it is easier to produce a longer duty cycle when compressions are administered at a faster rate (Maier et al. 1984; Ornato et al. 1988).
Defibrillation and Cardioversion
In children with ventricular fibrillation or pulseless ventricular tachycardia, the immediate management should be defibrillation, without delay to secure an airway.
Electric Countershock
Electric countershock, or defibrillation, is the treatment of choice for ventricular fibrillation and pulseless ventricular tachycardia. Defibrillation should not be delayed to secure an airway, because the likelihood of restoring an organized rhythm decreases with increased duration of fibrillation. Ventricular fibrillation is terminated by simultaneous depolarization and sustained contraction of a critical mass of myocardium (Zipes et al. 1975), allowing the return of spontaneous, coordinated cardiac contractions, assuming the myocardium is well oxygenated and the acid–base status is relatively normal. Drug treatment may be required as an adjunct to defibrillation, but by itself cannot be relied on to terminate ventricular fibrillation.
An older generation of defibrillator that is still present in many hospitals delivers energy in a monophasic damped sinusoidal waveform. This type of instrument delivers a single, unidirectional current with a gradual decrease to zero current. By contrast, the newer generation of biphasic defibrillator delivers a current in a positive direction for a set period, followed by a reversal in current. Biphasic defibrillators are more effective than monophasic defibrillators in terminating ventricular fibrillation in adults; therefore, their use is recommended where possible. If available, use of pediatric attenuator pads or a pediatric mode on the AED should be used in children 1–8 years of age, but if unavailable (and a standard defibrillator is similarly unavailable), an unmodified AED should be used.
In the majority of adult cases, energy levels of 100–200 J are successful when shocks are delivered with minimal delay (Weaver et al. 1982; Campbell et al. 1977). The goal of defibrillation is to deliver a minimum of electrical energy to a critical mass of ventricular muscle while avoiding excessive current that could further damage the heart. The most reliable predictor of success of defibrillation is the duration of fibrillation before the first countershock (Kerber and Sarnat 1979). Acidosis and hypoxemia also decrease the success of defibrillation (Kerber and Sarnat 1979).
Practical Aspects of Defibrillation in Children
Correct paddle size and position are critical to the success of defibrillation. The largest paddle size appropriate for the child should be used because a larger size reduces the density of current flow, which in turn reduces myocardial damage. In general, adult paddles should be used in children weighing more than 10 kg, and infant paddles should be used in infants weighing less than 10 kg. Paddle force is important as well. If the entire paddle does not rest firmly on the chest wall, a current of increased density will be delivered to a small contact point. Paddles should be positioned on the chest wall so that the bulk of myocardium lies directly between them. One paddle is placed to the right of the upper sternum below the clavicle; the other is positioned just caudad and to the left of the left nipple. For children with dextrocardia, the position of the paddles should be a mirror image. An alternative approach is to place one paddle anteriorly over the left precordium and the other paddle posteriorly between the scapulae.
The interface between the paddle and chest wall can be gel pads, electrode cream, or electrode paste. The electrode cream produces less impedance than the paste. Electric current follows the path of least resistance, so care should be taken that the interface material from one paddle does not touch that of the other paddle. This is especially important in infants, in whom the distance between paddles is small. If the gel is continuous between paddles, a short circuit is created, and an insufficient amount of current will traverse the heart. The use of bare metal paddles increases the risk of arcing and worsens cutaneous burns from defibrillation. The use of self-adhesive pads is preferable when feasible.
In an oxygen (O2)-enriched atmosphere, sparking from poorly applied defibrillator paddles can cause a fire. Therefore, any sources of free-flowing O2 should be removed to a distance of at least 1 m from the child. These potentially hazardous O2 sources include nasal cannula O2, “blow-by” O2, and nebulizers powered by O2. By contrast, O2 in a closed circuit may remain on the child. For example, it is not necessary to disconnect the ventilator from the child’s tracheal tube (if a ventilator is disconnected, the fresh gas flow should be turned off because large volumes of O2 flow through disconnected ventilators).
For the first defibrillation attempt, 2 J/kg of delivered energy should be administered. After shock delivery, CPR should resume immediately with chest compressions for five duty cycles (2 min). If one shock fails to eliminate ventricular fibrillation, the incremental benefit of another immediate shock is small. Resumption of CPR is likely to confer a greater benefit than another shock. CPR may provide coronary perfusion, increasing the likelihood of defibrillation with a subsequent shock. It is important to minimize the time between chest compressions and shock delivery and between shock delivery and resumption of postshock compressions (Kleinman et al. 2010). Approximately 2 min of CPR should be delivered before a second attempt at defibrillation at twice the original energy level (4 J/kg) (Kleinman et al. 2010).
If ventricular fibrillation or pulseless ventricular tachycardia persists beyond the second defibrillation attempt, standard doses of epinephrine should be administered (with subsequent doses every 3–5 min during persistent cardiac arrest). After 2 min of chest compressions, defibrillation should be attempted again, followed by administration of amiodarone (5 mg/kg) or lidocaine (1 mg/kg) with subsequent defibrillation attempts. It is not necessary to increase the energy level on each successive shock during defibrillation after the second dose. However, successful defibrillation has been reported with currents in excess of 4 J/kg without adverse sequelae, up to a maximum dose not exceeding 10 J/kg or the adult level, whichever is less (Kleinman et al. 2010). This sometimes occurs when a fixed energy level, adult automated external defibrillator (AED) is used in a small child.
Open-Chest Defibrillation
If the chest is already open in the OR or easily opened as in a fresh postoperative cardiac patient, ventricular fibrillation should be treated with open-chest defibrillation, using internal paddles applied directly to the heart. These should have a diameter of 6 cm for adults, 4 cm for children, and 2 cm for infants. Handles should be insulated. Saline-soaked pads or gauzes should be placed between the paddles and the heart. One electrode is placed behind the left ventricle and the other over the right ventricle on the anterior surface of the heart. The energy level used should begin at 5 J in infants and 20 J in adults.
Automated External Defibrillation
The use of AEDs is now a standard therapy in out-of-hospital resuscitation of adults (Berg et al. 2010; Weaver et al. 1982). AEDs are now deemed appropriate for use in children older than 1 year. If available, the use of pediatric attenuator pads or a pediatric mode on the AED should be used in children 1–8 years of age, but if unavailable (and a standard defibrillator is similarly unavailable), an unmodified AED should be used.
Transcutaneous Cardiac Pacing
In the absence of in situ pacing wires or an indwelling transvenous or esophageal pacing catheter, transcutaneous cardiac pacing (TCP) is the preferred method for temporary electrical cardiac pacing in children with asystole or severe bradycardia. TCP is indicated for children whose primary problem is impulse formation or conduction, with preserved myocardial function. It is most effective in those with sinus bradycardia or high-grade atrioventricular block, with slow ventricular response but adequate stroke volume. TCP is not indicated for children during prolonged arrest, because in this situation, it usually results in electrical but not mechanical cardiac capture, and its use may delay or interfere with other resuscitative efforts.
To set up pacing, one electrode is placed anteriorly at the left sternal border and the other posteriorly just below the left scapula. Smaller electrodes are available for infants and children, but adult-sized electrodes can be used in children weighing more than 15 kg. ECG leads should be connected to the pacemaker, the demand or asynchronous mode selected, and an age-appropriate heart rate used. The stimulus output should be set at zero when the pacemaker is turned on and then increased gradually until electrical capture is identified on the monitor. After electrical capture is achieved, whether an effective arterial pulse is generated must be determined. If not, additional resuscitative efforts should be initiated.
The most serious complication of TCP is the induction of a ventricular arrhythmia. Fortunately, this is rare and may be prevented by pacing only in the demand mode. Mild transient erythema beneath the electrodes is common. Skeletal muscle contraction can be minimized by using large electrodes, a 40-ms pulse duration, and the smallest stimulus required for capture. If defibrillation or cardioversion is necessary, a distance of 2–3 cm must be allowed between the electrode and paddles to prevent arcing of the current.
Vascular Access and Monitoring During Cardiopulmonary Resuscitation
Vascular Access and Fluid Administration
One of the key aspects of successful CPR is early establishment of a route for administration of fluids and medications. If intravenous access cannot be established rapidly, the intraosseous or endotracheal route should be used.
Endotracheal Medication Administration
In the absence of other vascular access, medications including lidocaine, atropine, naloxone, and epinephrine (mnemonic LANE) can be administered via the endotracheal tube (Ward 1983; Johnston 1992). The use of ionized medications such as sodium bicarbonate or calcium chloride is not recommended by this route. The peak concentration of epinephrine or lidocaine administered via the endotracheal route may be less compared with the intraosseous route. For example, the peak drug concentration of epinephrine after endotracheal administration was only 10 % of that after intravenous administration in anesthetized dogs. The recommended dose for epinephrine via the endotracheal tube is ten times the intravenous or intraosseous dose or 0.1 mg/kg for bradycardia or pulseless arrest.
The volume and the diluent in which the medications are administered through an endotracheal tube may be important. When large volumes of fluid are used, pulmonary surfactant may be altered or destroyed, resulting in atelectasis. The total volume of fluid delivered into the trachea with each drug administered should not exceed 10 mL in children and 5 mL in infants and neonates (Greenberg et al. 1981). However, administering an adequate volume of a drug is important to reach a large area of mucosal surface beyond the tip of the endotracheal tube for absorption. Absorption into the systemic circulation may be further enhanced by deep intrapulmonary administration by passing a catheter beyond the tip of the tracheal tube deep into the bronchial tree. The risk associated with the endotracheal route of drug administration is the formation of an intrapulmonary depot of drug, which may prolong the drugs’ effect. This could theoretically result in postresuscitation hypertension and tachycardia or the recurrence of fibrillation after normal circulation is restored.
Monitoring During Cardiopulmonary Resuscitation
A basic clinical examination is vital during cardiac arrest. The chest is carefully observed for adequacy of bilateral chest expansion with artificial ventilation and for equal and normal breath sounds. In addition, the depth of compression and the position of the rescuer’s hands should be constantly reevaluated in performing chest compressions by palpation of a major artery. Palpation is essential in establishing the absence of a pulse and in assessing the adequacy of blood flow during chest compressions. Palpating the peripheral pulses may be inaccurate, especially during intense vasoconstriction associated with the use of epinephrine.
An indwelling arterial catheter, when available, is a valuable monitor in assessing the arterial blood pressure. Specific attention should be paid to diastolic blood pressure as it relates directly to adequacy of coronary perfusion during CPR. In addition, arterial access allows for frequent blood sampling, particularly for measurement of arterial pH and blood gases. Pulse oximetry can be used during CPR to determine the O2 saturation and may be of value in assessing the adequacy of cardiac output, as reflected in the plethysmograph. The ECG can suggest metabolic imbalances and diagnose electrical disturbances.
The ETCO2 monitor provides important information during the course of resuscitation. Leading resuscitation organizations strongly recommend the use of capnography to confirm correct placement of the endotracheal tube, to monitor the quality of CPR, and to provide an early indicator of ROSC (Deakin et al. 2010). Because the generation of exhaled CO2 depends on pulmonary blood flow, it can provide a useful indicator of the adequacy of cardiac output generated by chest compressions. As the cardiac output increases, the ETCO2 increases and the difference between end-tidal and arterial CO2 becomes smaller (Tang et al. 1991). In animal models, ETCO2 during CPR correlates with coronary perfusion pressure and with ROSC (Sanders et al. 1989; von Planta et al. 1989). A reduced ETCO2 may occur transiently in the presence of adequate chest compressions after administration of epinephrine owing to an increase in intrapulmonary shunting. The International Liaison Committee on Resuscitation (ILCOR) in its international consensus (2010) acknowledges the fact that low values of ETCO2 are associated with low probability of survival, and the committee believes however that there are insufficient data to support or refute a specific cut-off of ETCO2 as a prognostic indicator of outcome during adult cardiac arrest. Touma and Davies (2013) did a systematic review studying the prognostic value of end-tidal carbon dioxide during cardiac arrest. The ability of ETCO2 cut-off values to reliably predict the outcome of resuscitation with high accuracy is not established. ETCO2 monitoring may be considered to evaluate quality of chest compressions, but there is no pediatric evidence that ETCO2 monitoring improves outcomes from cardiac arrest (de Caen et al. 2015).
Temperature should be monitored during and after CPR. The resuscitation of the child with hypothermia as the cause of cardiac arrest must be continued until the child’s core temperature exceeds 95 °F (35 °C). A glass bulb thermometer measures the temperature to very low values. Repeated measurements of core body temperature should be made at several sites (rectal, bladder, esophageal, axillary, or tympanic membrane) where possible, to avoid misleading temperature readings from a single site, because local body temperature may vary with changes in regional blood flow during CPR. Hyperthermia should be aggressively treated in the peri-arrest period, because postarrest hyperthermia is associated with worse outcomes in children (Hickey et al. 2000). Evidence suggests a benefit to induced hypothermia after resuscitation from cardiac arrest in adults (Hypothermia After Cardiac Arrest Study Group 2002; Bernard et al. 2002) and after perinatal hypoxic or ischemic injury (Shankaran et al. 2002). The data available to support the use of hypothermia in infants and children after cardiac arrest is from case series and retrospective studies. In the recently published Therapeutic Hypothermia After Pediatric Cardiac Arrest (THAPCA) study, therapeutic hypothermia, as compared with therapeutic normothermia, did not confer a significant benefit with respect to survival with good functional outcome at 1 year (Moler et al. 2015).
Medications Used During Cardiopulmonary Resuscitation
α- and β-Adrenergic Agonists
In 1963, only 3 years after the original description of closed-chest CPR, Redding and Pearson (1963) demonstrated that early administration of epinephrine in a canine model of cardiac arrest improved the success rate of CPR. They also demonstrated that the increase in aortic diastolic pressure with the administration of α-adrenergic agonists was responsible for the improved success of resuscitation. They theorized that vasopressors such as epinephrine were of value because the drug increased peripheral vascular tone and, hence, coronary perfusion pressure. The relative importance of α- and β-adrenergic agonist actions during resuscitation has been widely investigated. In a canine model of cardiac arrest, only 27 % of dogs that received a pure β-adrenergic receptor agonist along with an α-adrenergic antagonist were resuscitated successfully, compared with 100 % of dogs that received a pure α-adrenergic agonist and a β-adrenergic antagonist. Other investigators have demonstrated that the α-adrenergic effects of epinephrine resulted in intense vasoconstriction of the resistance vessels of all organs of the body, except those supplying the heart and brain (Michael et al. 1984). Because of the widespread vasoconstriction in nonvital organs, adequate perfusion pressure and thus blood flow to the heart and brain can be achieved despite the fact that cardiac output is very low during CPR (Michael et al. 1984; Koehler and Michael 1985; Schleien et al. 1986).
The increase in aortic diastolic pressure associated with epinephrine administration during CPR is critical for maintaining coronary blood flow and enhancing the success of resuscitation (Niemann et al. 1985; Sanders et al. 1984). Even though the contractile state of the myocardium is increased by the use of β-adrenergic agonists in the spontaneously beating heart, β-adrenergic agonists may actually decrease myocardial blood flow by increasing intramyocardial wall pressure and vascular resistance during CPR (Downey et al. 1979). By its inotropic and chronotropic effects, β-adrenergic stimulation increases myocardial O2 demand, which, when superimposed on low coronary blood flow, increases the risk of ischemic injury.
Any medication that causes systemic arterial vasoconstriction can be used to increase aortic diastolic pressure and resuscitate the heart. For example, pure α-adrenergic agonists can be used in place of epinephrine during CPR. Phenylephrine and methoxamine, two α-adrenergic agonists, have been used in animal models of CPR with success equal to that of epinephrine. Their use results in a greater O2 supply to demand ratio in the ischemic heart and at least a theoretical advantage over the combined α- and β-adrenergic agonist effects of epinephrine. These agonists, as well as other classes of vasopressors such as vasopressin, have been used successfully for resuscitation.
The merits of using a pure α-adrenergic agonist during CPR have been questioned by some investigators. Although the inotropic and chronotropic effects of β-adrenergic agonists may have deleterious hemodynamic effects during CPR for ventricular fibrillation, increases in both heart rate and contractility will increase cardiac output when spontaneous coordinated ventricular contractions are achieved.
Epinephrine
Epinephrine (adrenaline) is an endogenous catecholamine with potent α- and β-adrenergic stimulating properties. The α-adrenergic action increases systemic and pulmonary vascular resistance, increasing both systolic and diastolic blood pressure. The increase in diastolic blood pressure directly increases coronary perfusion pressure, thereby increasing coronary blood flow and increasing the likelihood of ROSC (Niemann et al. 1985; Sanders et al. 1984). The β-adrenergic effect increases myocardial contractility and heart rate and relaxes smooth muscle in the skeletal muscle vascular bed and bronchi. Epinephrine also increases the vigor and intensity of ventricular fibrillation, increasing the likelihood of successful defibrillation (Otto and Yakaitis 1984).
Larger than necessary doses of epinephrine may be deleterious. Epinephrine may worsen myocardial ischemic injury secondary to increased O2 demand and may result in postresuscitative tachyarrhythmias, hypertension, and pulmonary edema. Epinephrine causes hypoxemia and an increase in alveolar dead space ventilation by redistributing pulmonary blood flow (Tang et al. 1991; von Planta et al. 1993). Prolonged peripheral vasoconstriction by excessive doses of epinephrine may delay or impair reperfusion of systemic organs, particularly the kidneys and the gastrointestinal tract.
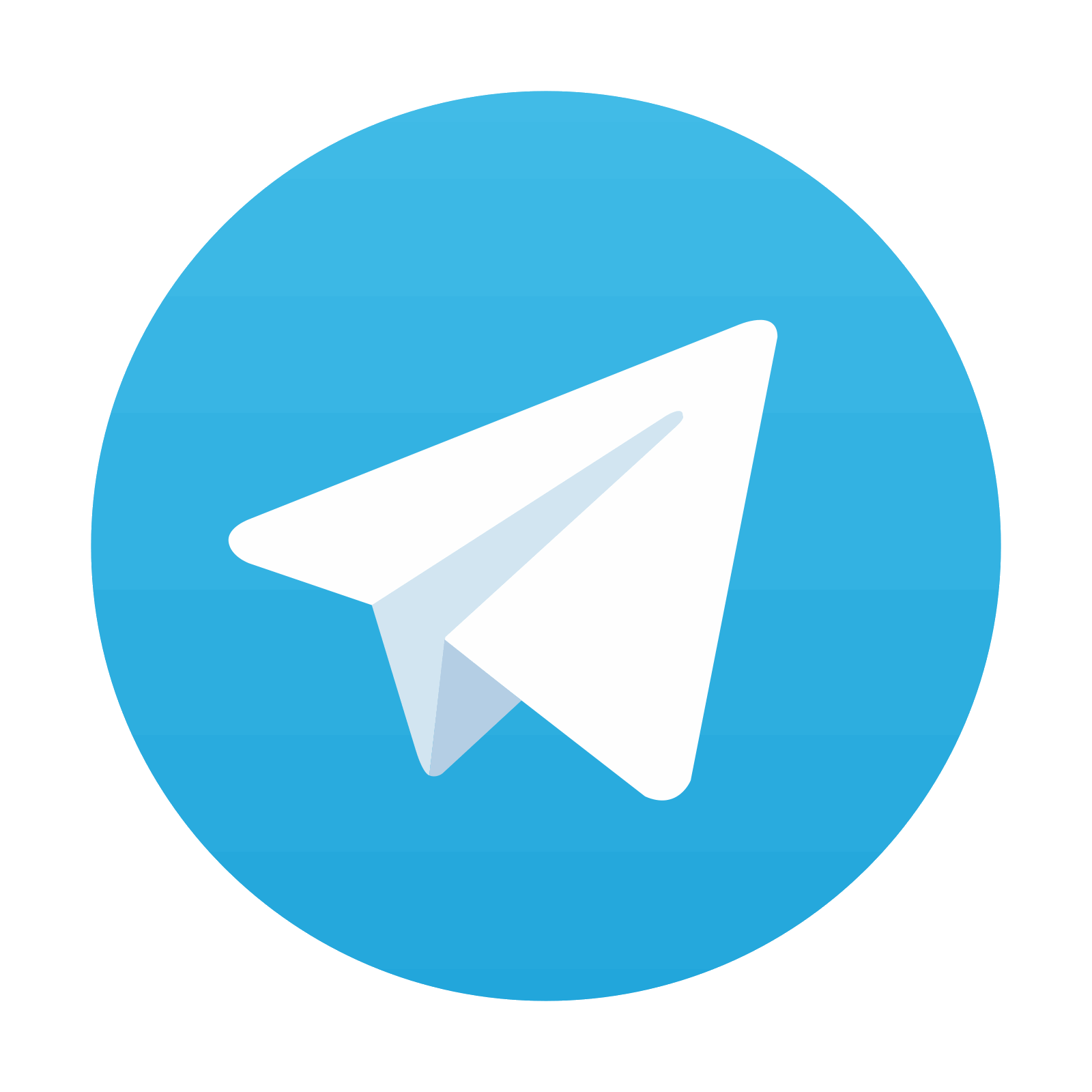
Stay updated, free articles. Join our Telegram channel
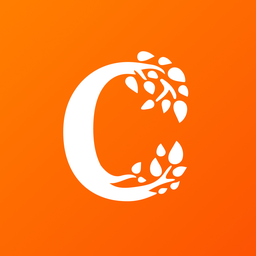
Full access? Get Clinical Tree
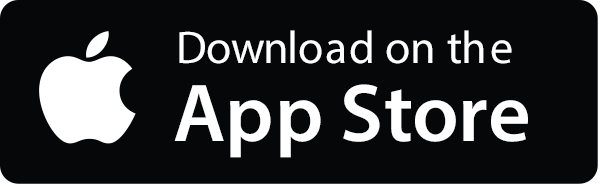
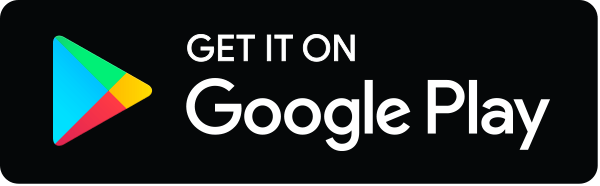