Circadian Rhythms and Sleep Biology
Sleep and circadian rhythm are highly coupled processes. In the original formulation they were considered independent but interacting. Borbely et al.1–4 posited that the circadian process (Process C) had a 24-hour rhythm that interacted with the sleep drive system (Process S) (Fig. 12-1). Process S is envisaged to be like an old-fashioned egg timer. The drive for sleep is at a very low level following the major sleep bout and increases progressively as wakefulness proceeds, that is, the drive to sleep is related to the duration of prior wakefulness. Humans are programmed to sustain wakefulness for 16 hours but beyond this develop progressive performance impairments. During the day the drive to sleep is counteracted by an alertness signal from the clock. When this alertness signal declines later in the evening, the sleep drive is unopposed and sleep ensues. During sleep the drive to sleep progressively declines, that is, the egg timer is flipped and the sands recover (Fig. 12-1). The situation is actually more complex than this since sleepiness occurs twice a day, that is, siesta time in early afternoon and late in the evening. While these processes were initially considered independent, they are not at a molecular level.5,6 Core clock molecules increase their expression in brain when sleep is deprived.7 Moreover, mutations of a clock-associated gene – DEC2, now called BHLEH41 – result in short sleep in humans (<6 hours) without evidence of daytime performance impairment.8,9
Figure 12-1 Two process model of sleep/wake control. Behavioral state is controlled by the interaction between two processes: (a) Process C, the biological clock and (b) process S, the sleep homeostatic drive. The dark bar below from 23:00 to 07:00 hours is the lights-off period. For further details, see text. (Reproduced with permission from Kryger MH, Roth T, Dement WC, eds. Principles and Practice of Sleep Medicine, 5th ed. Philadelphia, PA: Elsevier; 2011.)
In this chapter we describe the basis of the clock and make the point that the lung itself has a clock. We then describe sleep and review recent evidence that sleep alters gene transcription in the lung. The reader will get a background in sleep that will facilitate understanding the cardiopulmonary changes during sleep (see Chapter 101) and sleep-disordered breathing (see Chapter 99).
MOLECULAR MECHANISMS OF THE CLOCK
The fundamental principles of how the clock ticks are conserved across species, although the specific details, that is, genes involved, vary between species (for reviews, see10,11).
The original concept that the clock involves a distinct molecular mechanism came from identification of mutant fruit flies (Drosophila) with long circadian periods, short circadian periods, and flies with no circadian rhythm.12 Subsequently it was determined that these different flies all had different mutations in the same gene which was given the name period (PER), that is, the first clock molecule identified.13 Later forward genetic studies identified another clock molecule in Drosophila, that is, timeless.14 The first mammalian clock gene was also identified by forward genetic studies, that is, studying mice that had received a chemical mutagen – ENU – and had an abnormal circadian period.15 This clock molecule is Clock and is a transcription factor.
In both Drosophila and rodents the major mechanism of oscillation is a negative feedback loop (for mammalian model, Fig. 12-2). There are also positive feedback loops. In mammals the main clock proteins are the three-period proteins (PER1, PER2, and PER3) and the two cytochrome proteins (CRY1 and CRY2). These proteins form a complex in the cytoplasm that enters the nucleus where they inhibit their own transcription that is controlled by the CLOCK/BMAL1 complex (Fig. 12-2). The degradation of the PER and CRY proteins is important in setting the period of the clock. The PER proteins are phosphorylated by enzymes such as casein kinase 1e and CKII. Once phosphorylated, the PER proteins are targeted for ubiquitination and degradation. In humans mutations in PER2, in the site in which it is phosphorylated16 and also in CK1 delta,17 lead to familial phase advance syndrome (for reviews, see18). Individuals with this have marked phase advance going to sleep at 7:30 PM and waking up at 4:00 AM.
Figure 12-2 The major mechanisms that result in diurnal oscillation of clock genes. For further details, see text. (Reproduced with permission from Buhr ED, Takahashi JS. Molecular components of the mammalian circadian clock. Handb Exp Pharmacol. 2013;(217):3–27.)
The other core feedback loop involves the orphan nuclear-receptor genes—REV-ERBα/β and RORα/β. RORs activate BMAL1 while REV-ERBs repress BMAL1 and CLOCK RORs and REV-ERBs are themselves targets of CLOCK-BMAL1 and are negatively regulated by the repressors CRY1 and 2 and PER, particularly PER2.
Thus, the clock is a cell autonomous process and individual cells show circadian oscillation and clock mechanisms can be studied in vitro with cells in culture.
SUPRACHIASMATIC NUCLEUS—THE MASTER CLOCK
The master clock is in the suprachiasmatic nucleus (SCN) of the hypothalamus (for review, see19). It contains ∼20,000 neurons. Destruction of this nucleus by lesioning results in the animal becoming arrhythmic, that is, with no obvious circadian period.20,21 The intrinsic period of the clock is not exactly 24 hours and the clock is entrained each day to 24 hours by environmental cues. For the SCN, the main entrainment is by light/dark signals. There is a direct track from the retina to the SCN—the retinohypothalamic tract. Moreover, there is a separate light-sensing mechanism in the eye for entrainment of the clock. This was identified in knockout mice that lacked rods and cones in the retina. While they were blind, there was normal entrainment of the circadian system to light/dark.22,23 Subsequently, the basis of this light-sensing mechanism was identified (for reviews, see24,25). It is the pigment, melanopsin, that is in retinal ganglion cells scattered across the retina.26–28 This pigment is particularly sensitive to light in the blue color range.29 This has led to development of blue light boxes, blue light glasses, and glasses that filter out blue light to manipulate the light input to the clock. Following time zone change the output of the master clock is entrained to the new time zone by altered light/dark input. However, this reentrainment is relatively slow with the clock adjusting only about 1 h/d.
PERIPHERAL CLOCKS
Once clock molecules were identified, the surprising result was that they were expressed in all tissues (for review, see11,30). It is now realized that there are functioning clocks in all tissues. These peripheral clocks are synchronized with signals from the master clock in the SCN. These peripheral clocks result in rhythmic expression of genes in each tissue and of the order of 3% to 10% of all mRNAs show rhythmic expression in different issues.31 (The precise number of genes oscillating depends on the mathematical definition of oscillation such that some estimates of the number of mRNAs oscillating are higher than this.) Thus, there are substantial changes in the molecular functions of different organs at different times of day. The lung is no exception in this regard (see further below).
Peripheral clocks are not only controlled by signals from the master clock but also by signals that occur locally in the particular tissue (Fig. 12-3). Important signals include changes in autonomic nerve activity, body temperature, glucocorticoids, and feeding pattern (Fig. 12-3). Phase shifting of peripheral clocks occurs much more rapidly than for the SCN. Altering the feeding pattern of mice can produce large phase shifts in liver clocks without any effect on the rhythmic expression in genes in the SCN.32,33
Figure 12-3 There are peripheral clocks in all organs. This diagram is for liver, but there are also clocks in the lung (see text). The “master” clock is in the suprachiasmatic nucleus (SCN) of the hypothalamus. It sends signals to peripheral clocks to synchronize them. There is also local control of clocks by metabolites, etc. (Reproduced with permission from Mohawk JA, Green CB, Takahashi JS11. Central and peripheral circadian clocks in mammals. Annu. Rev. Neurosci. 2012;35:445–462.)
There is also a molecular clock, not surprisingly, in the lung (for review, see34). There is a clock in the Clara cells of the mouse bronchial epithelium.35 As in other organs, there are a large number of genes that show diurnal changes in expression including genes involved in extracellular matrix, cell cycle, and apoptosis.36 Genes encoding inflammatory molecules such as chemokine ligands also show diurnal oscillation.36 The immune response, which is such a critical function of the lung, is under circadian control.37 Circadian rhythm of other functions of the lung are known to occur, including in FEV1.38 Circadian variation also occurs in asthma symptoms38 as do changes in the cellular content of bronchoalveolar lavage fluids in patients with mild asthma.39
Although a new area of inquiry, there are already data that pathological processes may interfere with the normal clock mechanism in the lung. In rats ventilator-induced lung injury results in reduction of REV-ERBα mRNA and protein in lung.40 The role of REV-ERBα in clock mechanisms at the mRNA and protein level is increased in hyperoxic lung injury.41 The role of REV-ERBα in clock mechanisms was described briefly earlier. It is likely that in the future the role of clock genes and mechanisms in pathogenesis of lung disease will be further elucidated.
CLOCK GENES AND PHARMACOLOGY
Given that a large part of the genome is regulated by clocks, it is not surprising that clock mechanisms can affect actions of drugs (for reviews, see42,43). The absorption of many commonly used drugs show time of day effects as does drug metabolism and drug excretion.42,43 For example, there is a circadian variation in gene expression of several members of the cytochrome P450 system, the main system for drug oxidation, in liver.31 Protein levels of cytochrome P450 also oscillate across the day44 and there is a circadian rhythm of the activity of all cytochrome P450 enzymes.45
Thus, efficacy of specific drugs can vary with time of day. The anticoagulant effect of heparin, for example, varies with time of day.46 When heparin is administered by constant intravenous infusion, the anticoagulant effect varies at different times of day.46
The concept of chronopharmacology is most developed for cancer therapy. Levels of plasma 5-fluorouracil vary across the day when the drug is delivered at the same rate.47–49 There is a circadian variation of the pharmacokinetics of 5-fluorouracil.50 Clinical trials show that treatment efficacy and toxicity of a chemotherapeutic regimen for metastatic colorectal cancer are enhanced using appropriate chronotherapy compared to constant infusion.51
Thus, time of day circadian effects need to be considered in pharmacology. It is likely that there will be a developing interest in this aspect when considering optimal drug regimens.
SLEEP AND ITS STAGES
It is now realized that sleep is universal. While definitions of sleep in mammalian species are based on the electroencephalogram, behavioral criteria52,53 have led to identification of sleep in many species. In particular, a sleep state has now been identified in Drosophila,54 zebra fish,55,56 and most recently in the worm, Caenorhabditis elegans.57 Thus, all model systems are available to study sleep. There are already data that molecular mechanisms regulating sleep are conserved across species.58
While there is a suggestion that there are different stages of sleep in Drosophila,59 rapid eye movement (REM) sleep has only been recognized in mammals and birds. In mammals sleep is divided into two major types—nonrapid eye movement (NREM) sleep and REM sleep (for review, see60). REM sleep is a stage with the following: the brain is quite active with flurries of activity resulting in REMs; individuals waking from REM sleep recall their dreams; there is active paralysis of muscles apart from the diaphragm such that normal individuals do not live out their dreams. Individuals with REM behavior disorder do enact their dreams since the atonia of REM sleep is no longer in place. This is a synucleinopathy and a high percentage of individuals with REM behavior disorder go on to develop Parkinson disease (for review, see61). Also in REM autonomic instability with variations in heart rate and breathing pattern (for further details, see Chapter 101). NREM sleep is divided into stages based on the electroencephalogram (Fig. 12-4). Originally four stages of NREM sleep were identified but recently stage 3 and stage 4 NREM sleep have been collapsed into one stage—N3. In this stage there is synchronized oscillatory firing of cortical neurons that generate large slow waves which are detected on the electroencephalogram (Fig. 12-4). In all mammalian species there is distinct cycling of the different sleep stages, although the periods of these cycles vary between species. In humans the cycles have approximately a 90-minute duration with episodes of REM sleep occurring every 90 minutes (Fig. 12-5). In the first part of the night individuals cycle down into stage 3 sleep; slow-wave sleep occurs early in the sleep period. Episodes of REM sleep get longer and indeed more phasic, that is, more eye movements etc., as the night progresses. Thus, typically humans are waking up out of REM sleep in the morning. There are major differences in the behavior of the cardiopulmonary system in these different sleep stages. These are discussed in Chapter 101.
Figure 12-4 Electroencephalographic tracings recorded from a normal young adult demonstrating the four stages of NREM sleep. In the stage 2 recording, the arrow points to a characteristic K complex and the underlining to sleep spindles.
Figure 12-5 The progression of sleep stages across a single night’s sleep of a normal young adult. The histogram was drawn on the basis of continuous recordings scored in 30-s epochs.
MECHANISMS CONTROLLING SLEEP
Sleep is a circuit property, that is, there is no evidence that single neurons sleep, although the intensity of “sleep” may vary across the brain, that is, local sleep slow waves in NREM sleep are particularly marked over brain regions that have been very active during wakefulness as a result of their involvement in specific tasks.62,63 Much work over the last two to three decades has identified the neuronal basis for sleep/wake control (for reviews, see60,64). Many neuronal groups have increased firing during wakefulness, reduced firing during NREM sleep, and virtually absent firing during REM sleep. These include the following: Cholinergic cells in basal forebrain; orexin (hypocretin) cells in lateral hypothalamus; histamine cells in posterior hypothalamus; dopamine cells in periaqueductal gray; noradrenaline cells in locus coeruleus; and serotonin cells in brain stem raphe nuclei. While these neurons are all more active in waking, they are likely to have different functions during wakefulness. For example, it has been shown that orexin (hypocretin) neurons respond to emotions, for example, positive emotions that result in pleasure increase the level of hypocretin.65
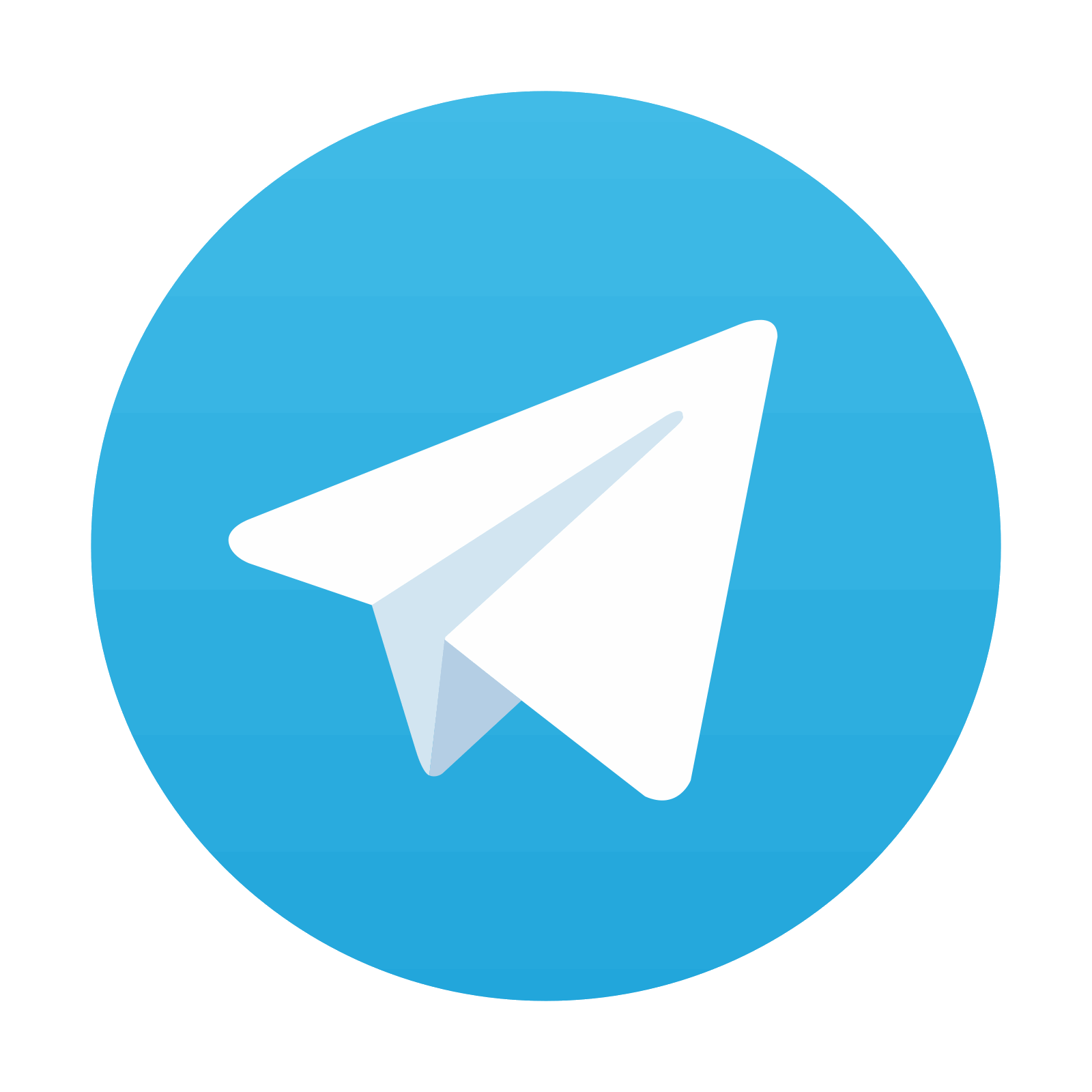
Stay updated, free articles. Join our Telegram channel
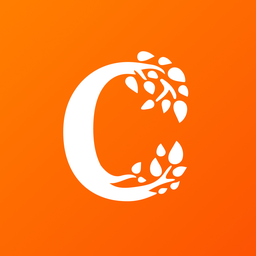
Full access? Get Clinical Tree
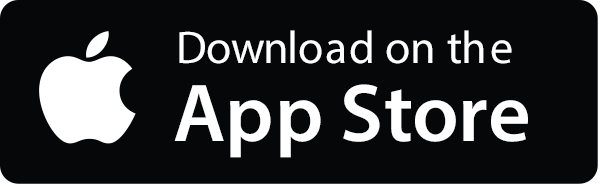
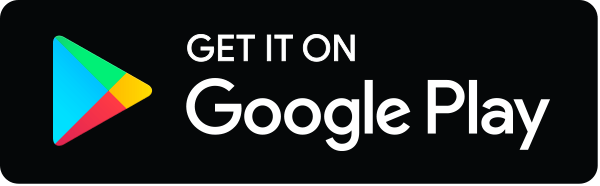