Changes in the Cardiorespiratory System During Sleep
As outlined in Chapter 12, sleep occurs in distinct states classified broadly as non-rapid eye movement (NREM) sleep and rapid eye movement (REM) sleep. These occur in a temporally organized fashion across the sleep period, with NREM dominating during the early hours of sleep and REM during the final hours of sleep. Myocardial infarction and ischemic stroke occur at a higher frequency during early morning hours,1–3 when REM sleep predominates. This temporal distribution of events may be explained at least in part by specific alterations in autonomic regulation during sleep. This chapter presents additional information on alterations in cardiopulmonary function during sleep.
CHANGES IN CARDIOVASCULAR CONTROL DURING SLEEP
During NREM sleep, heart rate slows by 5% to 10%,4 and blood pressure drops by about 10%.4 These changes are most prominent in the deepest stage of NREM sleep,4 that is, stage 4 (now known as N35 or slow-wave sleep4). The mechanism for this decline was elucidated by the seminal studies of Somers et al.4 using microneurography to record sympathetic bursts in the peroneal nerve in healthy humans during wake and in the different stages of sleep (Fig. 101-1). These studies confirmed a fall in sympathetic activity during NREM sleep, which is responsible for the drop in blood pressure, cardiac output, and systemic vascular resistance.4 This fall is accompanied by an increase in parasympathetic (vagal nerve) activity that is believed to be responsible for the bradycardia of NREM sleep.6 Thus, the balance of parasympathetic/sympathetic activity is altered during NREM sleep, with the parasympathetic being dominant. This also results in alteration in heart rate variability during sleep. The high-frequency component of this heart rate variability is said to reflect parasympathetic activity, while the low-frequency component is related to sympathetic activity.4 Thus, during NREM sleep there is an increase in the high-frequency component compared with wakefulness and a quite marked reduction in the low-frequency component of heart rate variability.4
Figure 101-1 Alterations in recorded bursts of sympathetic nerve activity (SNA) and blood pressure in wakefulness, different stages of non-rapid eye movement sleep (NREM) (stages 2–4), and rapid eye movement (REM). With deepening of NREM sleep, there is progressive loss of sympathetic activity, which is virtually absent in slow-wave sleep (stage 4). Sympathetic activity returns in REM but is highly variable. (Reproduced with permission from Somers VK, Dyken ME, Mark AL, et al. Sympathetic-nerve activity during sleep in normal subjects. N Engl J Med. 1993;328(5):303–307.)
Changes in REM sleep differ from those that occur during NREM sleep. During REM sleep, which occurs at approximately 90-minute intervals, there is a return of sympathetic activity such that heart rate and blood pressure return to wakefulness levels.4 This has been shown directly by recording sympathetic nerve activity (SNA) in humans (Figs. 101-1 and 101-2).4 Thus, during REM sleep the high- and low-frequency components of heart rate variability are the same as in wakefulness.4
Figure 101-2 Changes in recorded sympathetic nerve bursts in a healthy human during transitions between different sleep stages. There are more bursts, that is, more sympathetic activity, in REM sleep compared with stage 2 NREM sleep (top panel) and more activity in stage 2 sleep when it is transitional with many microarousals than when fully established (bottom panel). (Reproduced with permission from Somers VK, Dyken ME, Mark AL, et al. Sympathetic-nerve activity during sleep in normal subjects. N Engl J Med. 1993;328:303–307.)
During REM sleep there is also phasic activity that occurs in bursts. These phasic bursts of activity result in REMs and hence the name for the state. Phasic bursts of activity can lead to both brief, sudden increases in heart rate and blood pressure that are mediated by increases in sympathetic activity,4,6–8 which are similar to waking levels,4 and by sudden, baroreflex-mediated periods of decrease.6 These changes have pathophysiological significance. During surges of heart rate, there are also increases in coronary blood flow.7,8 But these can be mismatched such that the increased delivery of blood flow is insufficient to meet the extra myocardial demands consequent to the increase in heart rate. Moreover, in animal models of severe coronary stenosis, phasic decreases in coronary arterial blood flow are found when heart rate increases.7 Such changes may play a role in the known diurnal rhythm of timing of reported acute cardiac events in humans.1–3
Episodes of abrupt slowing of heart rate can also occur during REM sleep. In addition to baroreceptor-mediated decelerations, sudden slowing of the heart rate may be a result of reduction in central autonomic input to the heart, an increase in vagal tone, or both.9 At the extreme, brief episodes of asystole in phasic REM sleep have been described in otherwise healthy adults.10
CHANGES IN VENTILATION AND ITS CONTROL WITH SLEEP
As with the cardiovascular system, there are important changes in ventilation during sleep.11 These, too, are different in NREM and REM sleep.11,12 During NREM sleep ventilation declines. The various studies in this area have been summarized by Krieger et al.13 All studies have reported this decrease, although the magnitude of change varies from study to study (see summary in Fig. 101-3). In general, there is a decline in tidal volume while the change in respiratory rate is more variable (see Table 1 in Krieger J et al., 1990).13 Ventilation in REM sleep is also consistently less than in wakefulness; some studies report a small increase in ventilation in REM compared with NREM sleep (0.9%–7.1%), whereas other studies report a further decrease (–1.1% to –10.8%).13 This variability is likely related to the variability of ventilation in REM sleep itself. As in the cardiovascular system, there are changes in ventilation in association with the phasic events of REM sleep.14 Both acceleration and slowing of respiratory rate are found as are declines in ventilation. These effects seem to vary between subjects (Fig. 101-4),14 with some subjects showing large reductions in inspiratory flow and increased respiratory frequency during REMs and others showing only subtle changes.14
Figure 101-3 Percentage change in minute ventilation from wakefulness to NREM sleep in several different studies. There is some variation among studies in the magnitude of the drop in ventilation in NREM sleep, but all studies show a decline. (Data from Krieger J, Maglasiu N, Sforza E, et al. Breathing during sleep in normal middle-aged subjects. Sleep. 1990;13:143–154.)
Figure 101-4 Changes in respiration during REM sleep. The data shown are for two normal healthy adults. The top traces are right and left electrooculogram [EOG(R) and EOG(L)] that show phasic eye movements during REM sleep. The bottom traces are ribcage (RC) and abdominal (ABD) motion. The subject in the top panel (labeled High Responder) has a marked fall in ribcage and to a lesser extent abdominal motion in association with the eye movements. The subject in the bottom panel (labeled Low Responder) has little alteration in ventilatory movements during these phasic eye movements. (Reproduced with permission from Neilly JB, Gaipa EA, Maislin G, et al. Ventilation during early and late rapid-eye-movement sleep in normal humans. J Appl Physiol. 1991;71(4):1201–1215.)
The changes in NREM sleep in normal humans are largely the result of increases in upper airway resistance.15 This resistance progressively increases going from stage N1 to stage N3 NREM sleep (Fig. 101-5). This increase in resistance is associated with decrease in upper airway muscle activity in muscles such as the genioglossus16–20 and tensor palatini15 in the soft palate. During REM sleep, pharyngeal muscle activity is shut down via sleep-specific motor inhibition,18 predisposing individuals to obstructive sleep apnea.
Figure 101-5 Upper airway resistance increases progressively on going from wakefulness to the deeper stages of NREM sleep. (Data from Tangel DJ, Mezzanotte WS, White DP. Influence of sleep on tensor palatini EMG and upper airway resistance in normal men. J Appl Physiol. 1991;70:2574–2581.)
The neural control of upper airway motoneurons is complex and involves inputs from many premotor neurons originating in the forebrain, midbrain, pons, and medulla,21 which communicate with upper airway motoneurons via a diverse group of neurotransmitters. It has always been the hope that identification of the neurochemical change that is responsible for reduced upper airway motor tone would elucidate promising pharmacologic targets for obstructive sleep apnea. It is now clear that neurochemical drive to upper airway motoneurons varies with behavioral state and in fact is quite distinct in NREM sleep relative to REM sleep. In NREM sleep, reduced noradrenergic input from the pons16,19,20 contributes to reduced upper airway tone. Noradrenaline as a neuromodulator may play this role by potentiating glutamatergic excitation,16 rather than via direct excitation, as measured by masseter muscle activity. Muscarinic inhibition of the hypoglossal motor pool prevents the inhibition of genioglossus activity throughout REM sleep.18 During REM sleep, muscarinic receptors for acetylcholine linked to G-protein coupled, inwardly rectifying potassium (GIRK) channels have been shown to be important.18 Thus, preventing apneas in both NREM and REM sleep will likely require modulation of both noradrenergic and cholinergic neurotransmitters as well as potentially specific GIRK channels.
Individuals without upper airway obstruction as well as those with sleep-disordered breathing demonstrate increased PaCO2 in sleep. These findings support the concept that central ventilatory drive is also altered by sleep state. Although an increase in upper airway resistance is the major mechanism, it is, however, not the only mechanism, since even in laryngotomized subjects with tracheostomies, which bypass the upper airway, PCO2 increases in NREM sleep compared to wakefulness.22 Of interest, the effects of sleep on ventilatory drive are in part gender-specific. The relative importance of the increase in upper airway resistance reflects different neural control of upper airway muscles18–20,23,24 and the respiratory pump muscles such as the diaphragm. The former is much more coupled to state, that is, wake and sleep, while the latter, the diaphragm, is more affected by chemical control rather than variations in state (Fig. 101-6). This is why obstructive sleep apnea occurs commonly, whereas central sleep apnea is relatively rare. Sleep also alters the ventilatory response to hypoxia and hypercapnia. The ventilatory response to hypoxia declines in NREM sleep compared to wakefulness in men,25,26 but appears to remain the same in wakefulness and NREM sleep in women.27 This difference was attributed to higher rates of ventilation during wakefulness in men, with comparable rates in NREM sleep in men and women.27 The ventilatory response to hypoxia is lower in REM sleep than NREM in both men and women.25–27 Likewise, the slope of the ventilatory response to carbon dioxide is reduced in NREM sleep by as much as 50%,28 compared to wakefulness and further reduced in REM sleep. However, there is no compelling evidence that there is alteration in central neural response to chemical stimuli since there is, as outlined, a change in upper airway resistance that will alter the increase in ventilation produced by increases in the neural output to the diaphragm.
Figure 101-6 Schematic diagram illustrating the relative role of the “wakefulness drive” to breathe and that related to the chemical control system determined by PaCO2 and PaO2. The diaphragm is only little affected by the wakefulness drive (dashed line) and is predominantly responding to chemical stimuli (thick line).
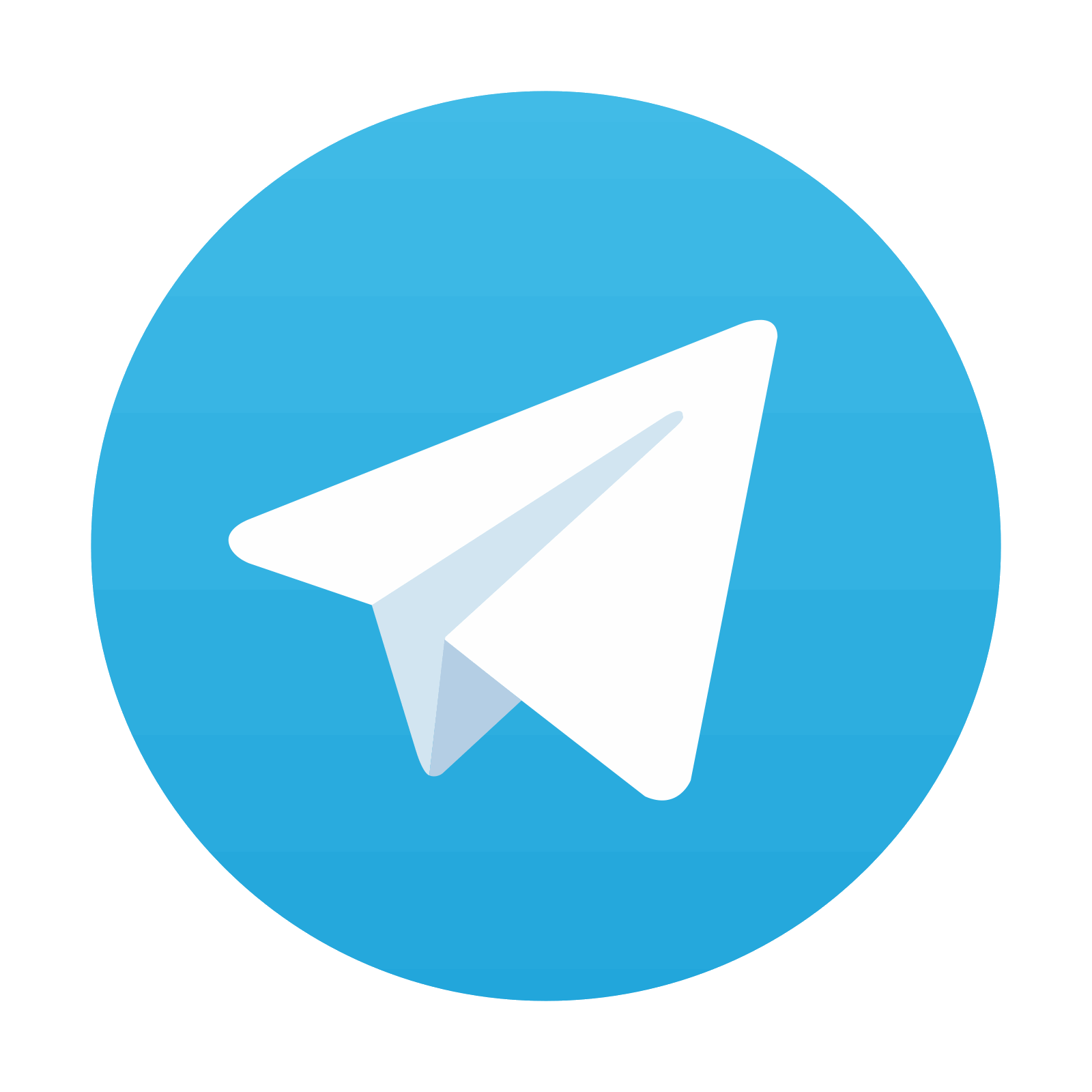
Stay updated, free articles. Join our Telegram channel
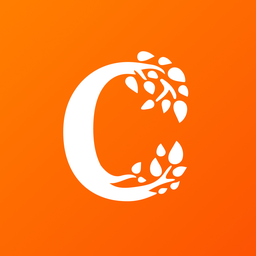
Full access? Get Clinical Tree
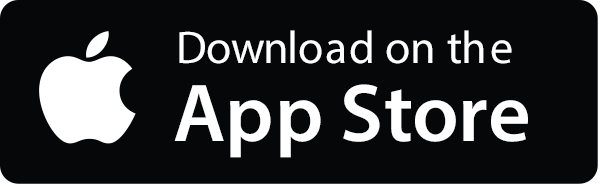
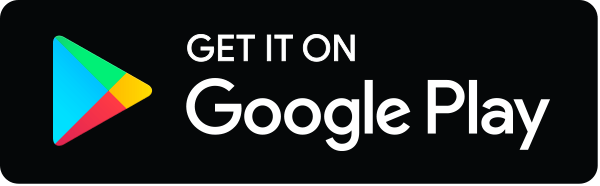