CHAPTER 62 Cardiopulmonary Bypass: Technique and Pathophysiology
Despite the belief that the need for CPB would be significantly diminished because of the surge in interest in off-pump coronary artery bypass grafting (CABG), the predictions have not materialized, and most institutions have reduced their use of off-pump CABG to only 10% to 15% of cases.1 In many practices, complex multiple arterial reconstruction and minimal-access surgery such as mini-thoracotomy mitral valve surgery are more comfortably and accurately approached using longer periods of CPB and techniques of myocardial protection. Therefore, we must be able to assess the impact of CPB on our patients. The surgeon must have a comprehensive understanding of all aspects of CPB, from the physiology of gas exchange to the molecular mechanisms characteristic of biocompatibility.
The evolution of CPB reflects ingenuity bred of necessity. A parallel discovery that contributed to the feasibility of extracorporeal circulation was the isolation of the natural anticoagulant heparin. Dr. John H. Gibbon, Jr. (Fig. 62-1), a “receptive and talented surgeon with foresight … who could envision an intact surviving patient beyond the isolated organ studies,”2 was the inventor of the Gibbons-IBM oxygenator. In 1953, this device was successfully used to correct an atrial septal defect. Although it was initially successful, three subsequent attempts at intracardiac repair were fatal, resulting in a self-imposed moratorium on its clinical use. Nevertheless, the feasibility of this approach had now been demonstrated.
The first clinically successful film and bubble oxygenators brought blood in direct contact with respiratory gases, to equilibrate with them. Complications related to blood trauma eventually led to a decline in their popularity, and they are rarely used today. Membrane oxygenators were introduced in the 1950s after the observation by Kolff and Berk3 that venous blood was oxygenated while flowing through a cellophane dialysis tube in contact with O2-containing dialysate. The first membranes were relatively impermeable to gases, requiring huge surface areas and massive priming volumes. Formed of silicone rubber, they were designed in either an extraluminal format (blood flowing on the outside of the tube with gas on the inside) or, less often, in an intraluminal format (the reverse). The refinements were subtle but significant, and we now have oxygenators with surface areas of 2.0 m2. The resulting minimization of prime volume has contributed more than any other factor to blood conservation practice in modern-day cardiac surgery.
TECHNICAL ASPECTS OF CARDIOPULMONARY BYPASS
Device Overview
Gravity drainage usually allows collection of blood from the venous circulation into the venous reservoir. Through separate inflows on the reservoir, blood can also be returned from the pericardial well (cardiotomy blood) and from cardiac and aortic vents. A centrifugal or roller pump is used to divert the venous reservoir blood to the oxygenator. After the blood passes through the integrated heat exchanger and oxygenator, it is circulated through an arterial filter and bubble trap and returned to the patient through the arterial cannula (Fig. 62-2). Cardioplegia setups are often intimately incorporated into the CPB circuit.
Principles of Current Oxygenator Design and Function
Diffusion of gases at the blood–membrane interface can be predicted by Fick’s law, which says that the rate of diffusion is proportional to the partial pressure gradient of the gas in the direction of diffusion. The rate of gas transfer is also inversely proportional to the distance through which it must pass (the thickness of the membrane), and it depends on the property of diffusivity of the membrane biomaterial. Currently used membranes are very permeable to O2, but they are often less permeable to CO2. The problem of poor diffusion of CO2 was solved by the introduction of microporous membranes. These surfaces allow transient direct blood–gas interfacing at pore structures smaller than blood cells; however, the hydrophobic nature of the membrane results in changes in blood surface tension, blocking direct contact between the two phases. As a result, the interface in microporous membranes behaves like a very thin stagnant film of plasma water that offers little resistance to gas exchange. Progressive protein accretion at the pores occurs over time, resulting in a finite functional capacity, detected by worsening efficiency in gas transfer, which is why oxygenators must be replaced with long CPB runs (Fig. 62-3).
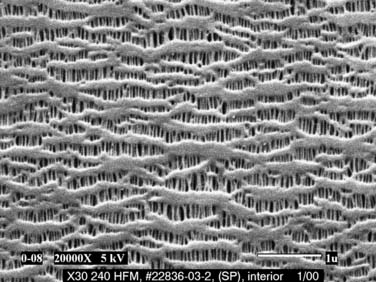
Figure 62–3 Scanning electron micrograph of a microporous membrane (Celgard) used in a membrane oxygenator (×20,000).
(Courtesy of Membrana GmbH, Germany.)
Hypothermia and Acid–Base Balance
The feasibility and applicability of hypothermia for heart surgery was first suggested by Bigelow and colleagues in 1950.4 They demonstrated the safety benefit of hypothermia as a means to decrease O2 consumption during inflow occlusion in an animal model. O2 consumption decreases by 50% for every 10° C drop in temperature (Fig. 62-4). Lower flows decrease collateral flow and rewarming of the heart from contact with adjacent tissues, and they provide a margin of safety if equipment fails.
Because alkalosis and hypocarbia trigger a decrease in cerebral blood flow (CBF), some investigators have suggested the addition of CO2 during hypothermia to compensate and to keep the pH unchanged (pH-stat strategy). In 1987, Murkin and coworkers5 confirmed that pH-stat management results in a greater ratio of CBF to cerebral metabolic rate (CMRO2) than alpha-stat management (i.e., no active correction of pH with hypothermia).5 Most agree that a pH-stat strategy is probably preferable in children, where increased CBF does increase the rate of cooling and thus increases the chance of achieving uniform cerebral hypothermia.6 The rate of brain oxygen depletion during deep hypothermic circulatory arrest is also considerably slower with pH-stat strategy. As a result, pH-stat substantially prolongs the interval between the onset of arrest and the exhaustion of brain oxygen stores and may be associated with better clinical outcomes in this group.7
In contrast, in adults, an alpha-stat strategy may provide greater cerebral protection during hypothermia on CPB. Alpha-stat management is certainly easier to accomplish, and a justification is that most cellular mechanisms are capable of maintaining intracellular pH despite fluctuations in extracellular conditions. Because excess CO2 is added in a pH-stat strategy, brain acidosis may occur during rewarming, and, combined with decreased O2 delivery after CPB, this may augment CNS injury in adults. Most importantly, as mentioned, a pH-stat strategy results in excessive CBF, which may increase embolic load. Finally, pH-stat strategy is associated with a decreased ability to maintain autoregulation at low pressures. Three randomized controlled trials have demonstrated a small but present benefit of alpha-stat strategy in terms of neurologic and neurocognitive outcome in adults, especially when CPB time exceeded 90 minutes.8
Hematocrit and Priming
The 1960s saw the introduction of crystalloid (dextrose 5% in water, or D5W) in prime as an alternative to routine whole blood prime. An increase in efficiency of oxygenation and a decrease in end-organ complications were seen with this approach.9 Now hemodilution is commonly practiced in virtually all adult and pediatric cardiac surgeries, and the hematocrit (Hct) is maintained between 20% and 25% during CPB. Hemodilution has a major effect on blood viscosity, primarily at the capillary level where the radius is small and the shear rate is low. Low flow at the capillary level increases viscosity of the blood, further increasing the resistance, but this effect is counter-balanced by the effect of hemodilution.
The optimal Hct for CPB remains a topic of significant controversy. A post-hoc assessment of the impact of low Hct on admission of cardiac patients to the intensive care unit (ICU) by Spiess and colleagues10 found an inverse correlation between admission Hct and the risk of Q-wave myocardial infarction (MI). On the other hand, studies have demonstrated that an Hct of less than 20% may be associated with abnormal distribution of blood flow to organs, and that an Hct of less than 15% may lead to maldistribution of coronary flow away from the subendocardium in the presence of residual coronary stenosis.11 Retrospective observational studies of consecutive patients undergoing isolated CABG have demonstrated that a lower minimum Hct is associated with a significantly increased risk of renal injury12 and mortality.13,14 As excessive hemodilution also increases CBF, it may cause a parallel increase in microembolization and thus may theoretically be a contributor to neurologic damage after CPB and the increased risk of stroke observed with lower Hct on CPB.15
Flow Rates, Perfusion Pressure, and Autoregulation
Flow is generally kept in the range of 2.2 to 2.5 L/min/m2 to provide a margin of safety during CPB, as systemic blood flow distribution and O2 consumption remain normal at this level. At normothermia, a target mean blood pressure of 50 to 70 mm Hg is generally used. The perfusionist can control the pressure by increasing or decreasing flow, or by the addition of vasoconstrictors or vasodilators (inhalational anesthetics). At lower temperatures, a mean pressure of 35 mm Hg is still generally accepted as safe. A number of investigators have recommended greater vigilance to prevent any hypotension (<45 to 50 mm Hg) during CPB,16 but these findings are controversial.17
Although the brain comprises only 2% of body weight, its metabolic needs demand 15% of the CO, extracting as much as 25% of the delivered O2. Temperature is the most important element influencing CBF during CPB. As the temperature is dropped, the CMRO2 decreases exponentially and the CBF decreases linearly (Fig. 62-5). As a consequence, the ratio of CBF to CMRO2 increases, resulting in “luxuriant” flow, further facilitated by hemodilution.
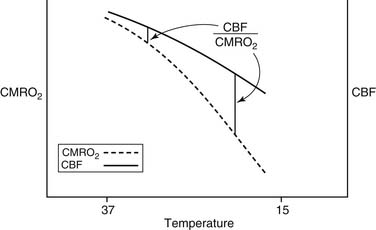
Figure 62–5 The effect of hypothermia on cerebral metabolic rate (CMRO2) and cerebral blood flow (CBF).
Autoregulation of CBF is also related to changes in perfusion pressure. At normothermia, a mean pressure of 50 mm Hg is the threshold at which the brain autoregulates flow, but with hypothermia (26° C), the threshold drops to 30 mm Hg (Fig. 62-6). At deep hypothermia (<20° C), there is a loss of pressure-flow autoregulation, as severe temperature reductions impair cerebral vascular relaxation,18 and changes in cerebral perfusion pressure alone result in corresponding proportional changes in CBF. Other factors that influence CBF and CMRO2 include blood viscosity, intracranial pressure and central venous pressure (CVP), and the blood gas status (pH, PaCO2, PaO2). CBF varies linearly with the PaCO2, in the range of 20 to 80 mm Hg, whereas a PaO2 of less than 50 causes cerebral vasodilation, which overrides pressure-flow autoregulation.
Pumps for CPB
The two commonly used types of pumps for CPB involve roller and centrifugal fluid propulsion. Noninterrupted contact of the rollers with the tubing in the track results in the nonpulsatile nature of the flow (Fig. 62-7A). A low compression will result in inadequate flow, whereas excessive compression may aggravate hemolysis and tubing wear. Other complications associated with the use of the roller pump include cavitation caused by excess pressure, and spallation (the release of particles from the inner surface).
Centrifugal pumps (see Fig. 62-7B) are a popular alternative to the roller pump, particularly in pediatric cardiac surgery and in cases with anticipated long CPB runs. The flow in a centrifugal pump is afterload dependent, and it is not predictable solely on the basis of the calculated revolutions per minute, so an in-line flowmeter is essential. Theoretically, its use should result in less blood trauma, particularly with prolonged CPB times, so this device is preferred for extracorporeal membrane oxygenation (ECMO). In children, the use of the centrifugal pump has been associated with decreased hemolysis, platelet activation, and inflammation and bleeding,19 although these findings have not been reproduced by other investigators.20 Aside from their expense ($150 per case), these devices are susceptible to air locks, thus requiring extreme vigilance by the perfusionist. On the other hand, this may be protective, as there is less chance of pumping large volumes of air into the patient via an inadvertent leak, and the lines cannot be over-pressurized with distal obstruction, as occurs with roller pumps. These devices are not valved, and if rotation stops without clamping the outflow, rapid retrograde flow from the arterial line occurs in milliseconds, essentially exsanguinating the patient. Finally, roller pumps can be operated manually, but a power outage with a centrifugal pump can be a disaster.
Cannulation for CPB
Arterial Cannulation
Oxygenated blood is returned to the arterial circulation through specially designed arterial cannulas, which come in a variety of configurations. Cannula characteristics include their length, the orientation of the tip (straight or right angle), the presence of a flange, and distal tapering. The arterial cannula is the narrowest portion of the CPB circuit after the oxygenator, and thus it is the site of the highest potential gradient. It has been demonstrated that gradients in excess of 100 mm Hg can be associated with hemolysis.21 There is no definite correlation between the French size of the cannula and the gradient, so investigators have derived alternative means to predict hemodynamics, such as the M number22 and the performance index.23 Finally, although the size and shape of the cannula have not been shown to influence the rate of transcranial Doppler-detected microemboli,24 design concepts, such as tips to diffuse the sand-blasting effect of flow, differential flow to the arch vessels, and distal baskets to capture debris, have been introduced to minimize (at least theoretically) the potential that the cannula may contribute to atheroemboli.
One of the most perplexing problems related to arterial cannulation is the management of patients with extensive aortic calcification. With bypass grafting, the use of off-pump surgery with a “no-touch” technique may be considered. Alternatively, femoral or axillary cannulation can be used with fibrillatory arrest, left heart venting, and arterial grafting. A third potential strategy involves the threading of a long cannula beyond the atheroma, into the descending thoracic aorta. Long cannulas are associated with a decreased peak, forward flow velocity, and turbulence compared with short cannulas.25 Borger and Feindel26 demonstrated that cannulation beyond the cerebral vessels with a long cannula decreased the count of cerebral emboli as detected by transcranial Doppler. Finally, in extreme cases, surgeons may cannulate the apex of the left ventricle with an armored venous cannula passed through the apex into the ascending aorta across the aortic valve. Epiaortic scanning has been advocated as a means to detect problems with the aorta and, when used consistently, may alter surgical management in terms of cannulation and clamp site as well as offering the option to proceed with off-pump surgery.27 This modality is effective in trained hands and more sensitive than epiaortic scanning and digital palpation.
PATHOPHYSIOLOGY OF CARDIOPULMONARY BYPASS
Noncellular Response
The pathophysiology of CPB relates to the unique responses that occur when the blood contacts the biomaterial surface (biomaterial-dependent processes) and non–contact-related processes such as cardiotomy blood collection and the effect of nonpulsatile flow. Within milliseconds of the blood’s contacting the synthetic surfaces of the CPB circuit, plasma proteins become adsorbed to the biomaterial. Although the amount, composition, and conformation of protein adsorption may differ between surfaces, there is no surface on which this process is completely inhibited, and each surface has a characteristic blood-adsorption pattern. Further exposure of the surface to blood results in activation of proteins of the contact-activation system (Fig. 62-8). This system comprises four primary plasma proteins: factors XII and XI, prekallikrein, and high-molecular-weight kininogen (HMWK). In the presence of the negatively charged biomaterial, a conformational change occurs in factor XII that permits its activation in the presence of prekallikrein and HMWK. Factor XIIa activates factor XI and initiates the intrinsic coagulation pathway, with the subsequent generation of thrombin and the cleavage of fibrinogen to produce fibrin, which is cross-linked by activated factor XIII. Factor XIIa also activates prekallikrein to form kallikrein within seconds of the start of bypass. Kallikrein catalyzes the conversion of HMWK to bradykinin and plays a role in the activation of the fibrinolytic system. Bradykinin has a very short half-life in the plasma because of its rapid metabolism by angiotensin-converting enzyme in the pulmonary circulation28 and by the vascular endothelium.29 It is believed to be a key mediator of increased capillary permeability and the development of tissue edema. Bradykinin mediates vasodilation by stimulating the release of endothelial nitric oxide,29 and it may also be an important mediator of cerebral ischemia.30
Activation of the fibrinolytic system during CPB is evidenced by increased levels of tissue plasminogen activator (tPA) and plasmin–antiplasmin complexes. Thrombin and bradykinin contribute to fibrinolysis through the direct activation of endothelial cells and the release of tPA, which further increases plasmin generation.31 Kallikrein-mediated activation of the fibrinolytic system includes its role in catalyzing the conversion of plasminogen to plasmin and the activation of pro-urokinase.32 Despite these findings, the contribution of fibrinolysis to post-bypass bleeding is controversial, as clot lysis activity subsides within minutes of terminating bypass.33 Another mechanism by which plasmin generation may contribute to bleeding is through its direct effect on platelet receptors during CPB.34
The complement system is activated through several mechanisms during CPB (Fig. 62-9). First, the third component of complement (C3), adsorbed to the CPB surface after releasing the potent chemoattractant C3a, is joined by the inactive factor B and properdin to produce the active proteolytic enzyme C3 convertase. C3 convertase cleaves the fifth component C5 to generate the active fragment C5a and the terminal complement complex C5b-9. Other mechanisms for complement generation through alternative pathways include the direct cleavage of C5 by kallikrein to produce C5a and the direct cleavage of C3 by plasmin. Complement generation has also been reported to be induced directly by endotoxin or directly by the cytokines tumor necrosis factor (TNF) and interleukin (IL)-6.35 Heparin–protamine complexes formed at the end of CPB activate the classical complement pathway, as does immunoglobulin bound on the biomaterial surface, which complexes with C1q.36
The generation of complement plays a key role in the recruitment of leukocytes, the upregulation of neutrophil activation markers, and the production of cytokines. Studies by Kirklin’s group37 confirmed that the incidence and degree of deranged function of the heart, lung, and kidney after CPB could be related to the raised plasma concentration of the complement fragment C3a. Furthermore, inhibition of production of terminal complement complex by a protease inhibitor was associated with a significant reduction in the deleterious effects of ischemia-reperfusion to the myocardium after CPB.38
Nitric oxide (NO) is a potent inflammatory mediator whose production is increased after CPB. Endogenously produced NO may cause tissue injury through formation of toxic peroxynitrites, activation of cyclooxygenase, and DNA deamination. Studies have reported a significant increase in inducible nitric oxide synthase (iNOS) expression in human lung after CPB, which may be related to cytokine release (e.g., TNF, IL-1, IL-6).39 This induction has also been shown to be associated with myocardial depression secondary to reperfusion injury.40
Finally, CPB initiates a cascade of events that results in cytokine release. This response may be triggered by changes in bowel mucosal blood flow and by bacterial translocation, as lipopolysaccharide (LPS) concentration increases by 100% immediately on CPB institution, with another significant increase seen after aortic cross-clamp release. LPS induces a broad range of immunologic effects and is considered the most potent stimulator of TNFα production from macrophages. TNF induces monocyte IL-1 production, and TNF and IL-1 in concert induce IL-6 production and release.41 Whereas a rise in TNF and IL-1 as a result of CPB has not been regularly demonstrated in all studies, a marked increase in IL-6 levels appears consistently with CPB. Peak concentration of IL-6 occurs a few hours after the end of CPB, with a gradual decrease toward preoperative levels in the following 24 hours,42 as seen in noncardiac surgery.43
Several investigators have demonstrated a correlation between the release of inflammatory mediators and myocardial dysfunction after coronary artery bypass surgery. Peak IL-6 and IL-8 levels correlate with the degree of myocardial dysfunction.44 TNF may be released locally in the myocardium, and this may be related to postischemic myocardial stunning.45 Jansen and coworkers46 demonstrated that a rise in TNF can be detected after release of the aortic cross-clamp. TNF, IL-6,47 and IL-8 levels correlated with the duration of the cross-clamping and the degree of myocardial injury as reflected by the creatine kinase (CK) MB isoenzyme levels.47
Cellular Activation during CPB: Platelets, Endothelial Cells, Leukocytes
The most consistently documented measure of CPB-related activation is an increase in the expression of guanosine monophosphate (GMP)-140 (P-selectin).48 Platelet glycoprotein (GP) IIb/IIIa is also likely activated,49 but it is controversial whether there is loss of other surface receptors such as GP Ib. Platelet microparticles, which may be highly procoagulant, can be consistently detected by flow cytometry after CPB.50 Other platelet products increased after CPB include β-thromboglobulin and platelet factor 4 (PF4).51
In clinical CPB, thrombocytopenia is seen commonly, with platelet counts dropping more than 50%, resulting not only from platelet adhesion to surfaces but also from hemodilution, platelet aggregate formation, and the formation of platelet–leukocyte complexes. With these changes, it is not surprising that the most predictable alteration in hemostatic function observed after CPB is platelet dysfunction. Clinical reflectors of this process include a universal prolongation of the bleeding time that is directly related to postoperative blood loss.52 Platelet aggregation to ADP and epinephrine is consistently abnormal,53 and there is decreased response to thrombin agonist receptor peptide (TRAP), suggesting a decrease in receptor sensitivity.54
Because of the intense anti-inflammatory reaction related to CPB, it might be expected that the endothelial layer would be at the front line of many of the cellular changes related to CPB. It is now evident that it is the activation of this axis that mediates many of the injurious processes related to CPB, such as reperfusion injury. The generation of cytokines is a major contributor to endothelial cell activation, primarily because they upregulate receptors necessary for neutrophil–endothelial cell binding.55 Endothelial cells undergo upregulation of intercellular adhesion molecule (ICAM) and E selectin. The latter binds to the integrin CD11a/CD18 present on resting polymorphonuclear leukocytes (PMN). PMN and monocytes may be activated with upregulation of the integrin CD11b/CD18 complex,56 which also binds ICAM on endothelial cells.57 The response to acute injury of the endothelium during CPB is evident by the acute rise in soluble P-selectin, with a concomitant fall in soluble E-selectin, and by increased elastase release.58 Leukocytes interact with platelets during CPB as another means of systemic inflammation. Increased GMP140 on the platelet surface is essential to mediate complex formation of platelets and monocytes or PNMs.57 Monocyte–platelet conjugates increased from 18% to 44%, whereas PMN–platelet conjugates increased only slightly.57
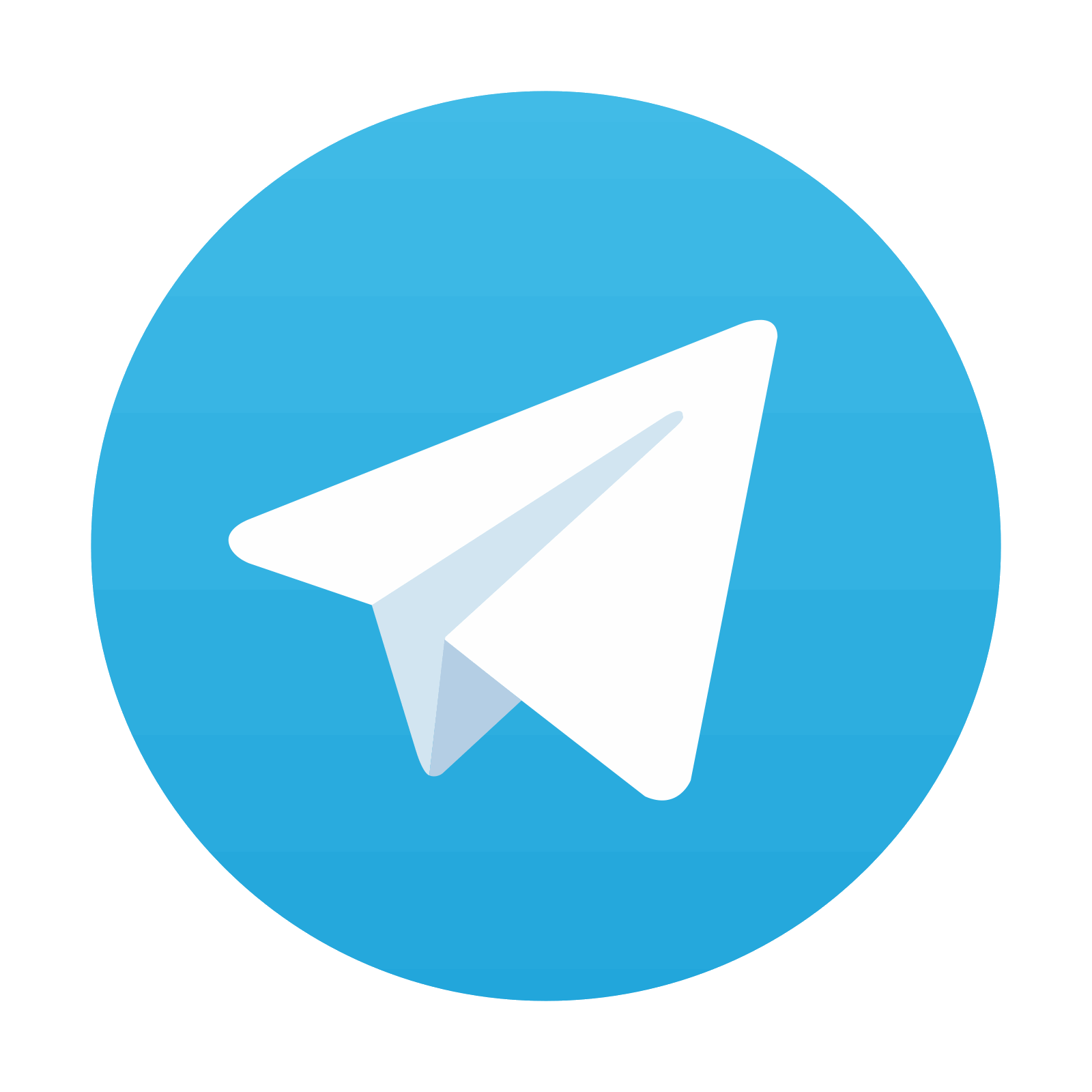
Stay updated, free articles. Join our Telegram channel
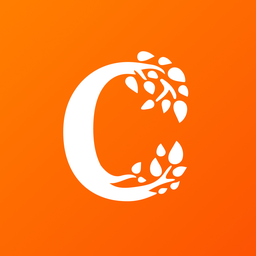
Full access? Get Clinical Tree
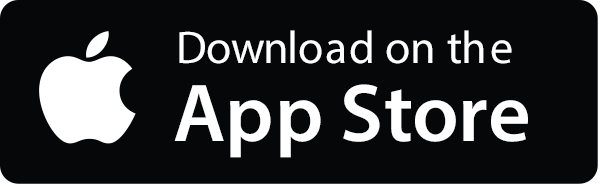
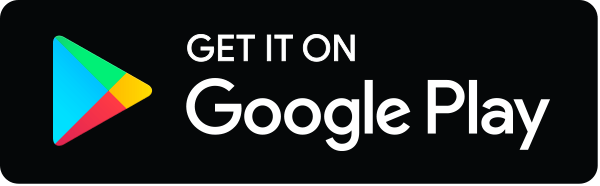