Chapter 42 Cardiac Neurotransmission Imaging
Positron Emission Tomography
THE CARDIAC AUTONOMIC NERVOUS SYSTEM
The autonomic nervous system, referred to as the visceral nervous system, consists of two divisions: sympathetic and parasympathetic innervation. The two systems differ in their major neurotransmitters—norepinephrine and acetylcholine—which define the stimulatory and inhibitory effects of each system.1 Sympathetic and parasympathetic innervation of the heart facilitates its electrophysiologic and hemodynamic adaptation to changing cardiovascular demands. Both sympathetic and parasympathetic tone control the rate of electrophysiologic stimulation and conduction, while contractile performance is primarily modulated by sympathetic neurotransmission. This functional characterization is reflected by the anatomic distribution of nerve fibers and terminals. Sympathetic fibers include multiple nerve endings that are filled with vesicles containing norepinephrine. They travel along epicardial vascular structures and penetrate into underlying myocardium much like coronary vessels. Based on tissue norepinephrine content, the mammalian heart is characterized by dense sympathetic innervation with a gradient from atria to base of the heart and from base to apex of the ventricles.2
In contrast to sympathetic nerve fibers, parasympathetic innervation is most prevalent in the atria, the atrioventricular (AV) node, and to a lesser degree within ventricular myocardium. Parasympathetic fibers in the ventricles appear to travel close to the endocardial surface, in contrast to sympathetic innervation. The enzyme choline acetyltransferase has been used as a reliable marker of cholinergic innervation.3 Choline acetyltransferase concentration is highest in the atria and decreases sharply in the right and left ventricular myocardium.
Figure 42-1 depicts neurotransmitter synthesis and turnover in the sympathetic and parasympathetic nerve terminal. Integrity and growth of both sympathetic and parasympathetic neurons are dependent on neurotrophins such as neuronal growth factor, which are secreted by myocardial target tissue and bind on nerve fibers via tyrosine kinase receptors, thereby inducing expression of growth-associated genes.4
Sympathetic Nerve Terminal
Norepinephrine, the dominant sympathetic transmitter, is synthesized from the amino acid tyrosine by several enzymatic steps. Generation of dopa from tyrosine is the rate-limiting step in catecholamine biosynthesis. Following dopa conversion to dopamine by dopa decarboxylase, dopamine is transported into storage vesicles by the energy-requiring vesicular monoamine transporter. Norepinephrine is synthesized by dopamine β-hydroxylase within storage vesicles. Nerve stimulation leads to norepinephrine release, which occurs as the vesicles fuse with the neuronal membrane and expel their content by exocytosis. Single nerve stimulation leads to exocytosis of only a small fraction of storage vesicles. The average adrenergic neuron has approximately 25,000 vesicles, with each containing approximately 250 pg of norepinephrine.5 Although most norepinephrine is thought to be released by exocytosis, nonvesicular release also occurs. Apart from neuronal stimulation, release is also regulated by a number of receptor systems. The α2-adrenergic receptors on the membrane surface provide negative feedback for exocytosis, which can thus be inhibited by presynaptic α2-agonists such as clonidine, guanabenz, and guanfacine.6 Muscarinic and adenosine receptors also have antiadrenergic effects. Neuropeptide Y is stored and released together with norepinephrine from the nerve terminal and is thought to inhibit neurotransmitter release.7 Presynaptic angiotensin II receptors and β-adrenergic receptors, on the other hand, mediate facilitation of norepinephrine release from sympathetic nerve endings so that antihypertensive agents such as angiotensin-converting enzyme (ACE) inhibitors or β-blockers can inhibit excessive norepinephrine release. The complex modulation of sympathetic neurotransmission6 obviously involves many systems, including dopamine, prostaglandin, and histamine. Only a small amount of norepinephrine release by the nerve terminal is actually available to activate receptors on the myocyte surface. Most norepinephrine undergoes reuptake into nerve terminals by the presynaptic norepinephrine transporter (uptake-1 mechanism) and recycles into vesicles or is metabolized in cytosol. The uptake-1 mechanism is characterized as saturable and sodium, energy, and temperature dependent.8 It can be inhibited by cocaine and desipramine.9 Structurally related amines such as epinephrine, guanethidine, and metaraminol are also transported by this system. In addition to neuronal uptake-1, the uptake-2 system removes norepinephrine into nonneuronal tissue.10 The uptake-2 mechanism is characterized as nonsaturable and not sodium, energy, or temperature dependent. It can be inhibited by, for example, steroids and clonidine.11 Free cytosolic norepinephrine is degraded rapidly to dihydroxyphenylglycol (DHPG) by monoamine oxidase (MAO). Only a small fraction of the released norepinephrine diffuses into vascular space, where it can be measured as norepinephrine spillover in coronary sinus vein blood.
Parasympathetic Nerve Terminal
Acetylcholine is synthesized within the parasympathetic nerve terminal. Choline is transported into the cytosol of nerve endings via a high-affinity choline uptake system,12 is then rapidly acetylated by choline acetyltransferase, and subsequently shuttled into storage vesicles. In contrast to amine uptake in sympathetic terminals, the choline uptake system is restrictive. Even close structural analogs are poor substrates for the high-affinity choline uptake system. Upon nerve stimulation, acetylcholine is released into the synaptic cleft, where it interacts with muscarinic receptors. Free acetylcholine is rapidly metabolized by acetylcholine esterase.
PET TRACERS FOR CARDIAC NEUROTRAMSMITTER IMAGING
Available tracers of sympathetic innervation differ in their specificity for uptake-1 and vesicular storage. Additionally, their usefulness is defined by the low complexity of radiochemical synthesis and by the resulting specific radioactivity and chemical purity. Low specific activity and/or chemical impurity result in concomitant exposure to nonlabeled catecholamines during tracer injection, which may compete with the radiotracer for specific uptake and storage and increase the likelihood of pharmacologic adrenergic effects. Table 42-1 summarizes currently available PET tracers for imaging of presynaptic adrenergic innervation. Specific tracers, which differ in their kinetic behavior (Fig. 42-2),13 are described subsequently in an order following the amount of published clinical experience.
Carbon-11-Hydroxyephedrine
Carbon-11 (13C)-labeled metahydroxyephedrine (mHED, or simply HED) is the most frequently used PET tracer for mapping of sympathetic neurons. It is synthesized by N-methylation of metaraminol, which reliably yields HED at high specific activity.14 HED is resistant to metabolism by MAO or catechol-O-methyltransferase (COMT) and has a high affinity to uptake-1. Nonspecific myocardial uptake is low, as demonstrated in isolated perfused rat hearts following desipramine blockade15 and in denervated human hearts early after cardiac transplantation.16 Although vesicular storage seems to occur, binding inside vesicles is reduced, owing to the higher lipophilicity of HED compared to norepinephrine.17 Addition of desipramine or norepinephrine to the perfusate following application of HED in isolated rat hearts resulted in accelerated HED washout, suggesting that cardiac HED retention is dependent on reuptake by the norepinephrine transporter. HED is thus believed to undergo continuous release and reuptake by sympathetic neurons.15
In contrast to single-photon emission computed tomography (SPECT) imaging using 123I-MIBG, where lower uptake in the inferior myocardium has been observed, distribution of HED throughout the left ventricular (LV) myocardium in healthy, normal individuals is regionally homogeneous, with high uptake in all myocardial segments (Fig. 42-3).16 For imaging, 400 to 700 MBq of 11C-HED are typically injected intravenously as a bolus, followed by a dynamic acquisition lasting 40 to 60 minutes. For quantification, a “retention index” is commonly calculated by dividing myocardial radioactivity concentration in the final image by the integral of the time-activity curve in arterial blood (which is derived from a region of interest in the LV chamber and needs correction for presence of radiolabeled plasma metabolites). Typical time-activity curves of dynamic HED PET studies are depicted in Figure 42-4. High neuronal affinity of HED makes compartmental modeling of its kinetics difficult, because in addition to norepinephrine transporter density, perfusion and transcapillary transport may significantly influence tracer uptake. An experimental, albeit complex, approach for truly quantitative kinetic modeling of HED has been introduced in the research setting18 but has not yet been transferred to human studies. In a rat model of varying degrees of cardiac sympathetic nerve denervation, semiquantitative measurement using HED retention was found to have a strong linear correlation with sympathetic nerve density, as reflected by cardiac norepinephrine transport (NET) density.19 Another experimental animal study aimed to investigate the feasibility of quantitation of 11C-HED PET retention in comparison to 123I-MIBG uptake by in vivo imaging. There was a close correlation between two quantitative parameters—myocardial 11C-HED retention fraction and the normalized 11C-HED activity (% ID/kg/g)—and 123I-MIBG uptake.20 These results would improve the understanding of the sensitivity of HED retention to denervation and aid in the interpretation of studies that use HED to investigate the effects of diseases on cardiac sympathetic innervation.
Hydroxyephedrine has been used in humans to identify alterations of sympathetic innervation in a number of pathophysiologic conditions, which are described in more detail later in this chapter. Additionally, HED has been used to determine effects of pharmacologic interventions on the sympathetic nervous system of the healthy heart. Prolonged impairments of myocardial catecholamine uptake following acute exposure to cocaine have been identified,21 and reduced myocardial HED retention was also observed in otherwise healthy chronic cocaine abusers.22 Further, the uptake-1 blocking effect of a novel antidepressant venlafaxine was characterized in a clinical trial in healthy volunteers.23
Fluorine-18-Fluorodopamine
Dopamine is a catecholamine that has high affinity for the dopamine transporter but similarly high affinity for the norepinephrine transporter. Fluorine substitution of dopamine has no significant effects on its biologic behavior,24 supporting the usefulness of fluorine-18 (18F)-fluorodopamine for evaluation of cardiac sympathetic nerves. For synthesis at sufficient specific activity, which is lower compared to, for example, 11C-HED, a complex no-carrier-added nucleophilic approach has been developed.25 Myocardial uptake of 18F-fluorodopamine occurs mainly via presynaptic uptake-1 and can be blocked by desipramine, as demonstrated in experimental26 and human studies.27 Following uptake into the neuron, fluorodopamine is sequestered into storage vesicles and β-hydroxylated to fluoronorepinephrine. Due to the half-life of 110 minutes for 18F, tracer clearance from the heart can be surveyed over a longer period than with 11C-labeled tracers (half-life, 20 minutes). Comparative studies of the kinetics of fluorodopamine and fluoronorepinephrine revealed a faster myocardial washout for fluorodopamine. These data suggested that myocardial radioactivity clearance after fluorodopamine injection can largely be attributed to inefficient β-hydroxylation and subsequent degradation by neuronal MAO.28 Additionally, rapid extraneuronal degradation has been observed. Soon after injection, radioactivity in blood corresponds mainly to radiolabeled metabolites.29
Because it permits measurement of tracer uptake and clearance, 18F-fluorodopamine has been proposed as a tracer of cardiac sympathetic innervation and function.26 It is the second tracer besides 11C-HED that has been used in a number of human studies to characterize cardiac innervation in disease conditions. For imaging, typically 35 to 140 MBq of 18F-fluorodopamine will be slowly infused over 3 minutes to avoid hemodynamic effects, then followed by dynamic PET acquisition over up to 3 hours. Because of the complex metabolic fate in myocardium and plasma, which renders quantitative kinetic modeling difficult, myocardial uptake and washout have mainly been described by basic calculations of regional radioactivity normalized to injected dose.
Carbon-11-Epinephrine
Epinephrine serves to a small degree as a physiologic neurotransmitter, but it is primarily a circulating catecholamine that is produced together with norepinephrine by the adrenal medulla and other chromaffin tissues. Epinephrine is radiolabeled with 11C by N-methylation of norepinephrine at high yield and high specific activity.3011C-epinephrine is handled in the myocardium in a manner similar to norepinephrine. It has high affinity for uptake-1, and although it is vulnerable to MAO degradation, efficient vesicular intraneuronal storage causes very slow clearance of radioactivity from the heart. In isolated perfused rat hearts, addition of desipramine to the perfusate following 11C-epinephrine administration (desipramine chase) results in much less acceleration of tracer washout compared to 11C-HED,31 suggesting that 11C-epinephrine is a tracer of neuronal uptake and vesicular storage, whereas 11C-HED largely reflects uptake-1 only. Plasma metabolism of 11C-epinephrine is considerable and needs to be taken into account for calculation of quantitative retention indices. In humans, only one study exists, that demonstrated that metabolite-corrected myocardial retention of 11C-epinephrine in healthy subjects was higher than that of 11C-HED, whereas denervated transplant recipients showed low levels of retention.32 It has thus been concluded that 11C-epinephrine may be more sensitive to neuronal abnormalities and, because of its more physiologic behavior, may be more suitable for evaluation of the cardiac presynaptic sympathetic nervous system.
Carbon-11-Phenylephrine
Phenylephrine is a sympathomimetic amine that is commonly used as a nasal decongestant. It can be labeled with carbon-11 at high specific activity.33 In contrast to HED and other catecholamine analogs used for PET imaging, phenylephrine is sensitive to MAO degradation. Studies in isolated perfused rat hearts have shown that 11C-phenylephrine is transported by uptake-1 at a slower rate than HED. Intraneuronally it is rapidly transported into storage vesicles, diffuses out of vesicles at a rate slower than HED, but is then metabolized by MAO in neuronal cytosol.34 Following injection of 11C-phenylephrine, there is thus considerable myocardial radioactivity washout, which is detectable during the imaging period (effective myocardial half-life approximately 60 minutes) and reflects MAO activity. 11C-phenylephrine may be combined with other short-lived neurotransmitter tracers such as 11C-HED and/or 11C-epinephrine to identify differential effects of disease on processes of norepinephrine uptake, vesicular storage, and metabolism in presynaptic adrenergic nerve terminals. Human experience with 11C-phenylephrine has been reported17 but remains limited.
Other Sympathoadrenergic Tracers
Similar to HED, metaraminol is a catecholamine analog that is resistant to metabolic degradation by MAO and COMT. Metaraminol can be fluorinated without altering the affinity to uptake-1. Myocardial accumulation in dogs correlated significantly with tissue norepinephrine content,35 and neuronal impairment induced by short-term ischemia was demonstrated.36 But the potent vasoactive properties of metaraminol and the limited specific activity following electrophilic labeling, which result in a mass of injected substance that is close to pharmacologic levels, have precluded clinical use of 18F-fluorometaraminol. Recently, however, efforts have been undertaken to develop alternative strategies for synthesis at higher specific activity,37 and the usefulness of fluorinated metaraminol may be reconsidered.
Efforts have also been made to label analogs of the SPECT tracer 123I-MIBG, with positron-emitting isotopes; 18F-parafluorobenzylguanidine, bromine-76 (76Br)-metabromobenzylguanidine, and 18F-fluoroiodobenzylguanidine have been synthesized successfully and tested in experimental settings.38–40 Kinetics of these tracers seemed to be comparable to those of 123I-MIBG, but affinity for uptake-1 was considerably lower than for classical PET tracers such as 11C-HED and 18F-fluorodopamine. Clinical applications of positron-emitting MIBG analogs for PET imaging have not yet emerged.
However, radiolabeled phenethylguanidines have recently been reinvestigated as a potential class of compounds with high vesicular retention. They are known to be even more potent depleters of cardiac norepinephrine stores than benzylguanidines, owing to their avid uptake and retention inside norepinephrine storage vesicles.41,42 For cardiac PET imaging studies, they can be labeled with 11C or 18F.42 Radiolabeling with 11C may offer some advantages over 18F in terms of being able to inject two to three times more activity into a patient because of 11C’s more favorable radiation dosimetry. This provides higher tissue and blood concentrations of the tracer early in the study when the kinetics of the tracer are changing most rapidly.42
Finally, the physiologic sympathetic neurotransmitter norepinephrine, has been fluorinated and used for PET imaging in baboons. It was shown that 18F-6-fluoronorepinephrine closely mimics the behavior of unlabeled norepinephrine.43 Synthesis yielded high specific activity, but experience remained limited to a few experimental studies, and the tracer has not yet entered human trials.
Tracers for Parasympathetic Innervation
Vesamicol is a compound that specifically binds to receptors on parasympathetic neuronal vesicles and inhibits storage of acetylcholine. Some vesamicol derivatives have been successfully labeled with positron-emitting radioisotopes, but most of them were tested for brain imaging; only 18F-fluoroethoxybenzovesamicol has been evaluated for cardiac imaging.44 In isolated perfused rat hearts, specific binding was low, consistent with low parasympathetic neuronal density, and nonspecific binding and washout were high. Additionally, there was considerable flow dependency of uptake, so that the usefulness of the tracer for clinical cardiac PET imaging was considered to be low.44
DISEASE-RELATED EXPERIMENTAL AND CLINICAL OBSERVATIONS
Coronary Artery Disease/Myocardial Infarction
Myocardial ischemia and infarction result in nerve injury.45 The sensitivity of cardiac sympathetic nerve terminals to ischemia has been supported by early experimental observations in dogs, where short periods of coronary occlusion resulted in sustained reduction of cardiac uptake of 18F-metaraminol.36 A first study in patients early after myocardial infarction (MI) by Allman and colleagues showed that the area of reduced HED retention exceeded the perfusion defect—a finding that was especially pronounced in non-Q-wave infarcts.46 Image examples of a patient after MI are shown in Figure 42-5. The hypothesis of a higher sensitivity of sympathetic neurons to ischemia compared to cardiomyocytes is further supported by observations of regionally reduced HED retention in the absence of resting perfusion defects in patients with advanced coronary artery disease (CAD) but no evidence of MI.47 Defects in HED uptake were also demonstrated in the chronically ischemic, hibernating myocardium in a pig model.48 A recent study combined 11C-HED and 11C-CGP12177 (nonselective hydrophilic β-adrenoceptor antagonist) PET to assess presynaptic and postsynaptic sympathetic nerve functions in patients with hibernating myocardium, as assessed by magnetic resonance imaging before and after revascularization. There were significant generalized (rather than regional) reductions in norephineprine uptake-1 mechanism and β-adrenoceptor density in these patients, indicating that increased sympathetic activity is a generalized phenomenon in chronic left ventricular dysfunction associated with CAD, rather than directly related to hibernation.49 The dependency of myocardial sympathetic innervation on myocardial perfusion and coronary flow reserve (CFR) was evaluated in nondiabetic CAD patients using 11C-HED, aiming to find a relationship between the coronary vascular reactivity and uptake. The degree of 11C-HED retention in viable myocardium of CAD patients did not correlate significantly with myocardial rest perfusion over a large flow range, but there was a correlation between regional 11C-HED retention and CFR. This indicates that sympathetic innervation can be preserved even when there is major impairment of resting myocardial blood supply.50 Probably the occurrence of denervation depends on the duration and severity of ischemic episodes in the presence of reduced CFR.50
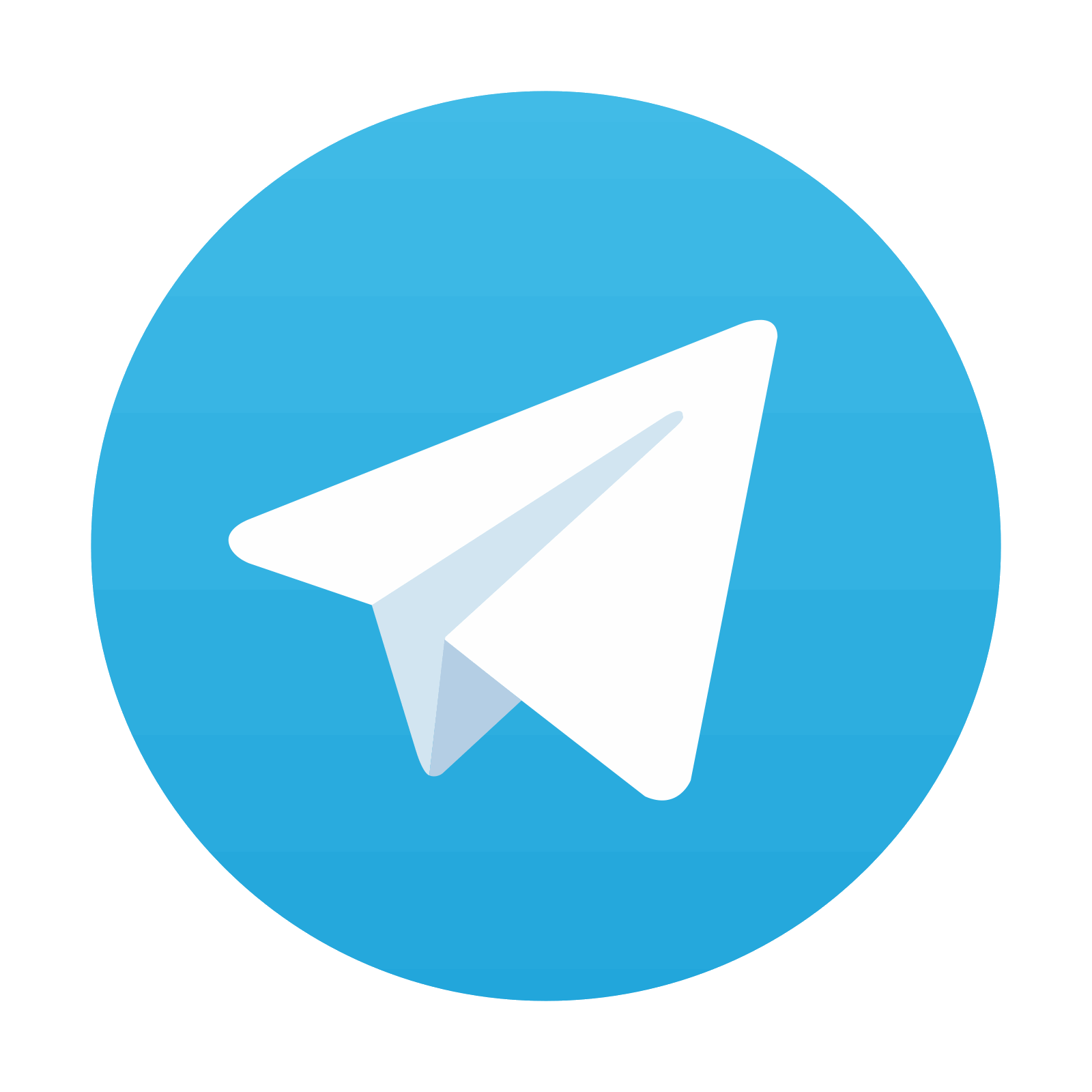
Stay updated, free articles. Join our Telegram channel
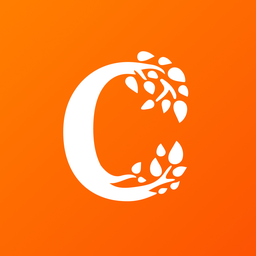
Full access? Get Clinical Tree
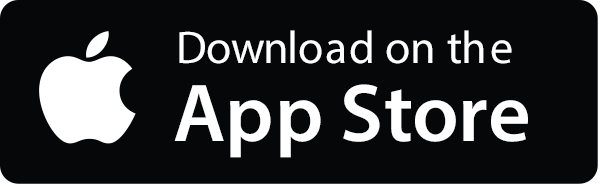
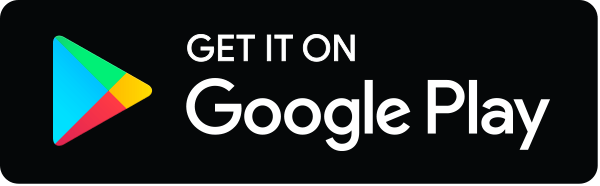