Cardiac Cell-Based Therapy: Methods of Application and Delivery Systems
Joshua M. Hare
Arnon Blum
Alan W. Heldman
The promise of cell therapy as a strategy to regenerate or repair myocardial tissue fulfills a true unmet need in cardiovascular (CV) medicine, and there is considerable interest in the potential of stem cells and other cell types to treat acute and chronic myocardial damage. Although major advances in medicine, interventions, and surgery have reduced all-cause mortality from CV disease, in the current era patients still endure morbidity and mortality from the irreversible injury caused by ischemic heart diseases. In this regard, revascularization (percutaneous coronary intervention or coronary bypass graft surgery) and medical therapy remain the mainstay of therapy of acute and chronic coronary artery disease, and medications such as 3-hydroxy-3-methylglutaryl coenzyme A (HMG-CoA) reductase inhibitors, angiotensin-converting enzyme inhibitors (ACEI), beta blockers, antiplatelet and anticoagulant therapies reduce patient risk and are effective at primary and secondary prevention. Despite these therapies, patients remain at risk for ventricular remodeling and heart failure after coronary ischemic events. Prevention or reversal of ventricular remodeling remains a major challenge in patient management. A number of lines of evidence now indicate that stem cell therapies may offer unique efficacy at reversing the myocardial scarring and adverse remodeling of ischemic heart disease.
A critical aspect in the developing field of cell-based therapy for heart disease is that of appropriate and adequate cell delivery. This important issue holds the promise of transforming the field of interventional cardiology, and in this chapter we review the emerging technologies and approaches to cell delivery.
STEM CELLS
Stem cells are defined by the ability to self-replicate and by the capacity to differentiate into a variety of lineages.1 Adult stem cells under active investigation for myocardial disease include bone marrow derived stem cells, circulating stem cells, cells from a variety of nonhematogenous sources, tissue-resident stem cells (cardiac stem cells), and pluripotent stem cells.
Stem cells from the bone marrow include mesenchymal stem cells (MSCs) and hematopoietic stem cells. From dozens of small and large animal models, and increasing numbers of human trials, it is becoming clear that stem cells have therapeutic potential after acute myocardial infarction (AMI) and in chronic myocardial dysfunction late after infarction. The mechanisms underlying cell therapeutics appear to be multiple, involving both direct cell actions (engraftment, differentiation into myocardial and vascular lineages) as well as indirect actions mediated in part by activating other cells, cell-cell interaction and paracrine pathways,2 cell fusion, and the stimulation and regeneration of resident cardiac stem cell niches.2
One strategy which may enhance the applicability of cell therapy is the concept of an “off-the-shelf” cell therapeutic, taking advantage of the immunoprivileged status of certain cell types, prototypically, MSCs which are both immunoprivileged and immunosuppressive.3 These stem cells can be cultured from healthy donors and expanded for storage and subsequent allogeneic use in a multitude of patients without the need for individual bone marrow aspiration in every
patient, and without the delay to treatment associated with cell expansion in vitro.
patient, and without the delay to treatment associated with cell expansion in vitro.
DELIVERY APPROACHES AND SYSTEMS OF DELIVERY
There is accumulating clinical trial evidence employing a variety of methods of cell delivery for at least four distinct clinical indications:
1. Acute myocardial infarction (AMI).
2. Chronic ischemic cardiomyopathy.
3. Hibernating myocardium.
4. Chronic ischemia leading to intractable angina (IA).
Delivery approaches or strategies include stimulating endogenous precursor cell recruitment, cell delivery via intracoronary, intravenous, or direct intramyocardial injection.
1. The systemic approach—granulocyte stimulating factor (G-CSF): A prototypic approach to stimulating endogenous precursor cells involves administering G-CSF to mobilize circulating CD34 cells such as endothelial precursor cells (EPCs). A large number of preclinical and clinical studies have tested whether G-CSF can be employed to stimulate the recruitment of endogenous cells4,5 to the myocardium. Animal studies and several pilot human studies have shown some evidence of reverse ventricular remodeling with the administration of G-CSF when given in the periinfarct period. However, larger randomized placebo-controlled studies showed no improvement in infarct size or ejection fraction in patients who had recent ST elevation MI.4,5 Studies have shown that intracoronary injection of G-CSF improved ejection fraction in patients with acute MI5, 6, 7 but data is conflicting with regard to chronic myocardial damage. G-CSF is in clinical testing currently to mobilize EPCs that are subsequently collected by apheresis and then readministered by intramyocardial injection in patients with chronic chest pain syndromes.4
2. The intracoronary route: Intracoronary delivery can be achieved with or without the use of a balloon occlusion catheter (the “stop flow” technique) to prevent backflow and prolong the residence of the administered cell suspension in the target coronary. Intracoronary administration has the advantage of targeting the ischemic and the periinfarction area by injecting the cells into the infarctrelated artery. This route is less invasive than surgery and easy to perform. Potential disadvantages include inefficient cell retention (washout) as well as microvascular obstruction, which has particularly been noted with MSCs3 or skeletal myoblasts.8 The direct intracoronary infusion is clinically used especially within 4 to 9 days after acute MI.9, 10, 11, 12, 13 The technique is similar to that used for coronary angioplasty, typically with the use of an over-the-wire angioplasty balloon inflated in the infarct-related stent. Coronary blood flow is then stopped for 2 to 4 minutes and stem cells are infused under pressure to the coronary artery of choice.14
3. The intravenous route: Intravenous administration is obviously a simple and low-cost route and has the potential to deliver cells to the largest population of patients. Disadvantages include early distribution to the lungs and poor myocardial retention. Peripheral venous infusion of stem cells (as performed in bone marrow transplants) represents a convenient way of delivering cells, and is based upon the principle that injury signals emanating from the damaged tissue recruit cells. A prototypic example is of SDF1α which binds to the CXCR4 receptors present on MSCs. A mouse model showed that transplanted human stem cells home to periinfarct areas15; however, only a few cells reached the affected area,16 and the general belief is that this technique could be applicable only after acute MI, because it relies on physiologic homing stimuli only. Another issue is the fact that many of the injected stem cells will be trapped in the microvasculature of the lungs, liver, and the lymphoid tissues.
4. Transendocardial route: There is now major interest in developing catheter systems for intramyocardial transendocardial injection of cells or other biologics for therapeutic purposes. The NOGA Myostar Catheter17 and the Biocardia Helical Infusion Catheter18 represent two injection systems that have been widely used in human studies. The transendocardial stem cell injection (TESI) strategy offers the ability to administer cells directly and accurately into the damaged myocardial segment without the need for surgery, avoiding the potential microvascular obstruction associated with the intracoronary route, and permitting access to any territory, even if its coronary supply is occluded and impassable. The risk of ventricular perforation appears to be low with proper case selection and careful technique. In some ways the technique of TESI is more akin to interventional electrophysiology than to the endovascular operations performed by most interventional cardiologists; catheter manipulation and navigation within the cardiac chamber may also be familiar to operators who had experience with investigation of catheter laser channel burning (so-called percutaneous myocardial revascularization, or PMR).19 TESI devices generally consist of several components, including a core element for cell transport, terminating distally in an injection needle. The core element may be advanced and retracted within the outer elements of the device. The support catheters are multifunctional, serving to protect the core and to direct it toward the regions of the myocardium to be targeted.
Four intramyocardial catheter-based delivery systems have been used in clinical trials. All share the design that was described, but differ in the anatomic approach to the myocardium. The Helix (BioCardia Inc., South San Francisco, CA, USA), the MyoCath (Bioheart Inc., Sunrise, FL USA), the Myostar (Biologics Delivery Systems, Diamond Bar, CA, USA), and the Stiletto (Boston
Scientific, Natick, MA, USA), all approach the myocardium from within the left ventricular (LV) chamber, with a transendocardial approach that is achieved by retrograde entry into the ventricle by crossing the aortic valve.
Scientific, Natick, MA, USA), all approach the myocardium from within the left ventricular (LV) chamber, with a transendocardial approach that is achieved by retrograde entry into the ventricle by crossing the aortic valve.
The MyoCath and the Myostar (Figures 36.1 and 36.2) are “integrated systems” where the core and support catheters are joined to form a single unit. These two devices are manipulated through a combination of axial rotation and deflection of the distal aspect (in a separate control mechanism capable of inducing up to 180° of flexion). While the tip of the device is in contact with the endocardium, the core catheter is advanced forcing a straight needle to a controlled intramyocardial depth of 3 to 8 mm. The integrated design provides an ability to navigate and to repeat injections; however, in both systems there is no guidewire lumen that may help in control and navigation, and they must be advanced from the femoral artery across the aortic valve using the same navigation mechanisms that guide the device within the ventricular chamber.19 The Helix and the Stiletto (Figures 36.3 and 36.4) are not integrated and the core catheter is a separate device that can be inserted and removed from the support catheters. The intraventricular manipulation and navigation are handled by a single, deflectable catheter (the Morph guiding catheter for the Helix infusion catheter) or by two preshaped support catheters (the Stiletto). Both systems are characterized by the ability to insert the support catheters into the ventricle over a guidewire and by the configuration of the injection needles (helical or spring loaded). In the helical design (reminiscent of a screw-in pacemaker lead) the needle tip may be more stable during the injection.
The Biocardia Helical Infusion System was used by us20 in porcine models and by our group and others for clinical trials.19 Its steerable deflectable guiding catheter allows access to nearly all parts of the left ventricle, and its helical screw-in needle provides positive engagement into the myocardium.21 In the spring-loaded needle device (the Stiletto) the needle is set to a fixed depth of 3.5 mm and may better penetrate fibrotic tissues. The Stiletto catheter is guided fluoroscopically18 in two planes. The Stiletto system allows rapid injection delivery to a number of endocardial sites. Neither the Helical infusion catheter nor the Stiletto system offers real-time assessment of the target myocardium, so that preinterventional imaging (with echocardiography, magnetic resonance, or computerized tomography) seems to be valuable for injection site selection. Nonetheless, careful and wellopacified biplane LV angiography, performed with an isocentered position, offers a wealth of information to the operator and may prove adequate for targeting. In preclinical experiments TESI is also feasible with realtime cardiac magnetic resonance imaging (MRI), which permits three-dimensional online assessments of the full thickness myocardium and perfusion.14
5. Epicardial delivery during surgery: Epicardial delivery was the first route of cell delivery that was tested. The advantage is the ability to inject the cells during heart surgery, although this fact is also the disadvantage of this method—it must coincide with a major operation.
6. Transcoronary-venous injection: The TransAccess Delivery System (Medtronic Vascular, Santa Rosa, CA, USA) (Figure 36.5) is a device that approaches the myocardium through the epicardial surface. A support catheter is positioned in specific branches of the cardiac venous system through the femoral vein. Guided by an intravascular ultrasound probe it is possible to localize the adjacent coronary artery and pericardium and to guide an injection catheter and needle into the myocardium through the epicardial surface.19 This delivery system has been used for skeletal myoblast delivery to scarred myocardium in patients with cardiomyopathy.22 Feasibility studies have shown a good safety profile for this technique. Infusion of stem cells through the coronary venous system (coronary sinus) under high pressure has been achieved in experimental models.23 The limitations include the lack of specific targeting and the variability and tortuosity of the coronary venous system.14
![]() Figure 36.2 The Myostar catheter. A. With NOGA mapping capability. B. Needle extension. C. Intramyocardial injection catheter with protruded needle. D. and E. control of the needle length. |
![]() Figure 36.3 The Helix catheter. A. Non-integrated system with independent deflectable guide catheter. B. Removable helical shaped needle injection catheter. |
![]() Figure 36.4 The Stiletto device. A nonintegrated system with 2 independent configured guide catheters and a removable spring-loaded needle. |
Which Route of Cell Delivery is the Best?
There is a limited dataset comparing methods of delivery, derived from experimental models, which to date do not uniformly support one approach over another. Perin et al. compared intracoronary (IC) versus transendocardial delivery in dogs with acute MI and found that transendocardial injections led to greater cell retention and a significant improvement in their LV ejection fraction compared with IC delivery.20 A small study that compared the transendocardial route to the IC and intravenous routes in ischemic swine heart found that the IC route delivered the highest amount of cells that were engrafted within the infarct zone. Engraftment of the cells delivered transendocardially was greater than the intravenous route delivery system. However, endocardial delivery had less engraftment to other organs other than the heart, and the IC route was associated with more microvascular obstructions.24 A study that compared transendocardial injection using the Boston Scientific Stiletto catheter with IC infusion (using an inflated occlusive balloon to deliver the cells into the distal bed) and with intravenous delivery of identically prepared MSCs in a porcine MI model24 found that this protocol of IC delivery was the most efficient technique; however, this technique caused microvascular obstruction in some animals. The preclinical studies suggest that infusing large numbers of MSCs directly into the coronary circulation could be dangerous because of possible occlusion.
Despite advantages and disadvantages, the delivery of MSCs into infarcted myocardium by transendocardial injection appears to be one of the most promising new approaches to cell-based therapeutics and is now supported by abundant preclinical studies and a growing clinical experience.
CELL TYPES USED FOR TRANSPLANTATION
Multiple candidate cells were suggested for regeneration of the injured heart, including embryonic stem cells,25, 26, 27 induced pluripotent stem cells,28 neonatal cardiomyocytes,29,30 skeletal myoblasts,31 EPCs,32 bone marrow mononuclear cells,9,33,34 MSCs,35 and cardiac stem cells.36,37
Embryonic stem cells have the capacity for self-renewal, can be clonally expanded, and are capable of differentiation into any cell type in the body.38,39 However, this potential could be dangerous, because they can form teratomas,40 they may induce immune rejection,40 and there is the ethical problem since they are created from early human embryos.41
Skeletal myoblasts have a contractile phenotype, can be used for autologous transplantation, and are resistant to ischemia. Several small nonrandomized phase I trials demonstrated a functional benefit, but with a high incidence of ventricular arrhythmias.42, 43, 44
Bone marrow derived cells are stem cells derived from the bone marrow. The relative ease in accessibility of bone marrow, and the large number of unfractionated autologous cells that can be obtained have been attractive for clinical use, and many human studies were done using these cells. They include rare hematopoietic, endothelial, and mesenchymal stem cells. Human hematopoietic stem cells can be defined as CD34+ cells capable of reconstituting all blood cell lineages45 and of transdifferentiating into cardiomyocytes, endothelial cells, and smooth muscle cells in vivo.46
Endothelial progenitor stem cells are hematopoietic cells that promote neovascularization directly47 or indirectly48 through secretion of proangiogenic cytokines.
MSCs are defined as CD105+ CD90+ cells, with a tendency to adhere to a plastic or tissue culture, and the capacity for multilineage differentiation including osteogenic, chondrogenic, and adipogenic lineages.49 They exhibit low immunogenicity50 and are attractive for stem cell therapy due to these traits. As donor cells they can be transplanted into recipients’ hearts to promote the restoration of cardiac function.51,52 In addition bone marrow MSCs have the capacity for angiogenesis, a trait that could enhance cardiac functional recovery.53,54 MSCs are rare, representing only approximately 0.01% of the bone marrow mononuclear cell fraction, but are attractive for therapy due to their low immunogenicity profile55,56 and the ability to expand many log-fold in vitro, allowing their use as an allogeneic graft.
Cardiac stem cells, the c-kit-positive, lineage-negative cardiac stem cells (CSCs) have been shown to improve postinfarction LV dysfunction in animal models. In a phase I trial (Stem Cell Infusion in Patients with Ischemic cardiOmyopathy [SCIPIO]) of autologous CSCs for the treatment of ischemic heart failure, post transplantation LV ejection fraction (LVEF) increased from 30.3% to 38.5% at 4 months (P = 0.001) in treated patients, while in controls LVEF did not change. At 1 year LVEF increased by 12.3 ejection fraction units versus baseline (P = 0.0007). In the seven treated patients in whom cardiac MRI could be done, there was a 24% decrease in infarct size at 4 months and a 30% decrease at 1 year.57
IMAGING FOR CATHETER GUIDANCE
Several approaches have been employed to help guide intramyocardial cell delivery. These include MRI, MDCT,58,59 and systems that integrate imaging guidance such as electroanatomic mapping.
Magnetic Resonance Imaging
MRI is emerging as highly valuable as it provides detailed information of cardiac anatomy that can help guide a TESI procedure. MRI enables precise delineation between viable and nonviable myocardium (i.e., that injured by MI). Thus, a myocardial injection procedure can be planned based upon integration of MRI images with conventional ventriculography (Figure 36.6).60,61
Cardiovascular magnetic resonance (CMR) is a rapidly evolving technology that is used for noninvasive imaging of the heart in different heart failure populations. CMR is performed at a magnetic field strength of 1.5 T. A CMR sequence generates its image by a series of radiofrequency pulses, magnetic gradient field switches, and timed data acquisitions. To prevent artifacts from cardiac motion most CMR images are gated to the R wave (of the ECG) and in order to overcome the respiratory motion CMR images are acquired in end expiratory breathhold.62
For infarct/fibrosis imaging gadolinium contrast agents are injected intravenously, and the areas that are detected with late or delayed (DGE) gadolinium enhancement are areas of scarring and fibrosis. The abnormally prolonged washout is due to a decreased functional capillary density in the irreversibly injured myocardium.63, 64, 65, 66 For perfusion imaging the dynamic passage of the contrast media (gadolinium) is followed through the cardiac chambers and the myocardium. Viability and post MI scarring are detected accurately, with highly specific patterns of fibrosis and scarring in ischemic and nonischemic cardiomyopathies.62
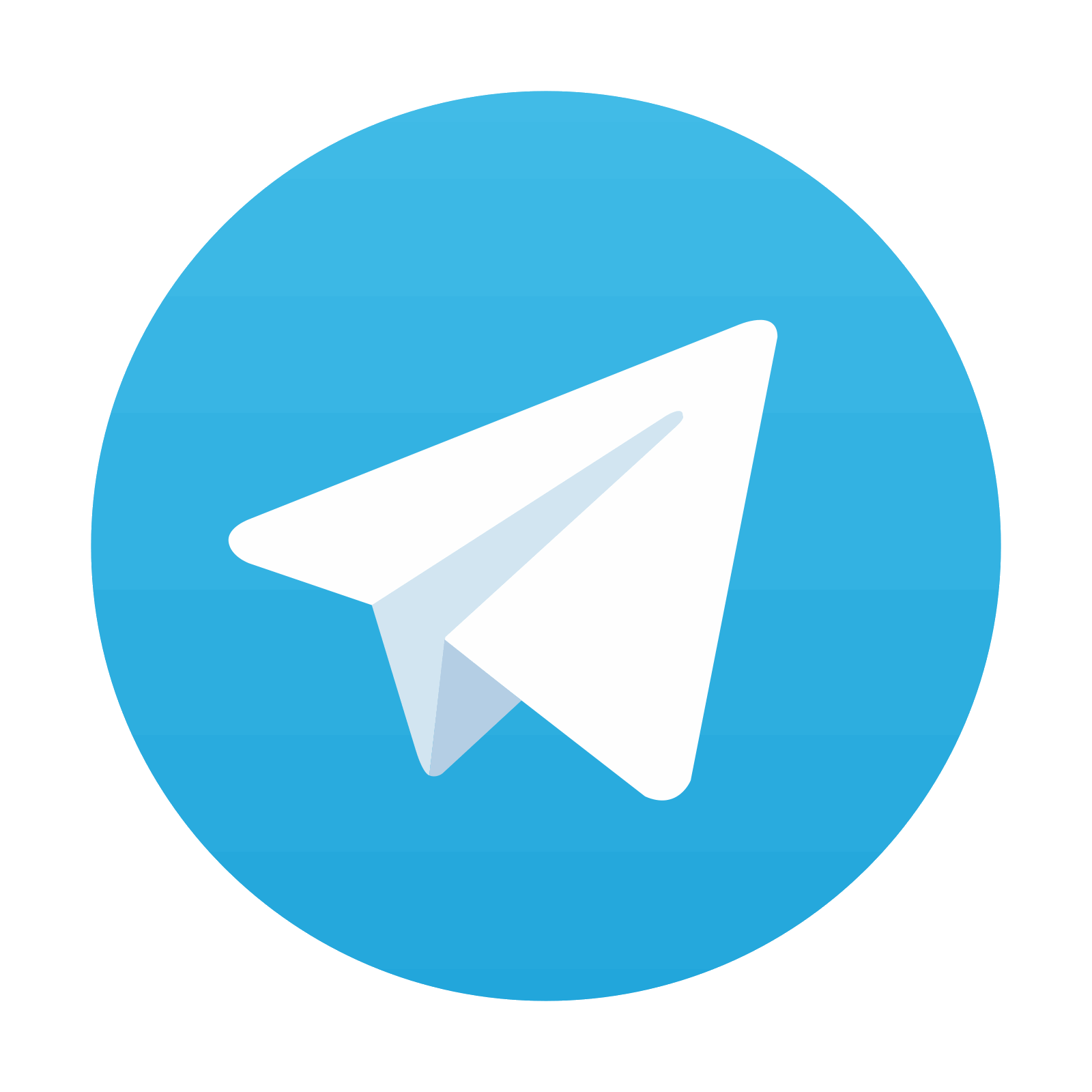
Stay updated, free articles. Join our Telegram channel
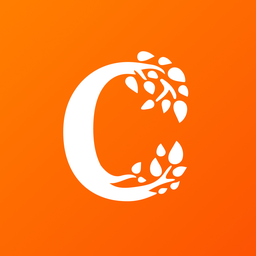
Full access? Get Clinical Tree
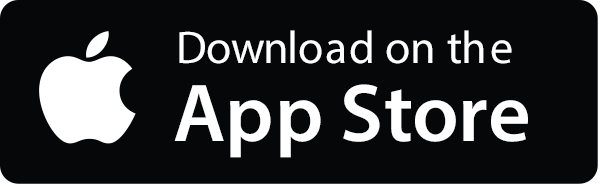
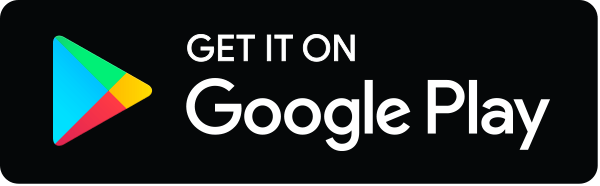