Chapter 1 Basic Electrophysiological Procedures for the Clinician
Electrophysiology Basic Concepts
All cells maintain electrochemical gradients across their membranes through the action of a panel of pumps, channels, transporters, and exchangers. The resulting voltage difference across the cell membrane is known as membrane potential (E). Although all cells maintain a resting membrane potential, excitable cells such as neurons and myocytes have the ability to generate transient, reversible, electrochemical wavefronts referred to as action potentials. Action potentials allow rapid signal propagation across the cell membrane. In the heart, each type of cardiac cell (e.g., nodal, atrial, ventricular, Purkinje) has a characteristic action potential that is determined by the panel of ion channels expressed (Figure 1-1, A and B).1,2 The action potential of the cardiac ventricular myocyte is composed of five phases (Figure 1-1, C).1 Phase 4 of the action potential is the resting membrane potential, which corresponds to cellular diastole. Phase 0 is the rapid depolarization phase driven by the influx of sodium (Na) ions. Phases 1 to 3 correspond to repolarization of the cell membrane. Phase 2, or the plateau phase, is maintained by the sustained influx of calcium ions and the efflux of potassium ions. This influx of calcium (Ca2+) activates Ca2+-induced Ca2+ release from the sarcoplasmic reticulum (SR), thereby activating myocyte contraction. Action potentials travel rapidly from cell to cell through low-resistance intercellular communication points called gap junctions. This behavior synchronizes electrical activation and facilitates coordinated muscular contraction.
The ability to study action potentials and ionic currents in biologic tissues was made possible by modeling the cell as an electrical circuit.3 The ionic current (I, measured in amperes) is the net flow of ions down their electrochemical gradients via their respective channels and transporters. The cell membrane, a lipid bilayer, serves as a capacitor (F, measured in farads), storing charge in the form of electrochemical gradients. The resistance (R, measured in ohms) to ionic current depends on many factors, such as the gating characteristics of ion channels, number of available channels, and posttranslational modification of channel proteins. The gating properties of ion channels vary, depending on specific channel type, but may be regulated by factors such as transmembrane voltage, ligands such as drugs, hormones or intracellular second messengers, mechanical forces, as well as the coexpression of regulatory subunits.4 Conductance (g), or the measure of ease of ionic current flow, is the inverse of resistance (g = 1/R). According to Ohm’s law, the membrane potential (E) equals the product of ionic currents (I) and channel resistances (R), E = IR. If conductance remained constant, the relationship between the current and the potential would be linear. However, because the conductance of ion channels is not constant, the resistor is non-Ohmic; therefore, the current-voltage relation is nonlinear. A simplified example of a cellular circuit is given in Figure 1-2.3,5
Electrophysiologic Tools
Experimental Preparations
Intact Animal
Study of cardiac electrical activity in a live animal is the most physiologically representative model system. All the available tools used in human electrophysiologic testing are readily available for animal studies as well. Intracardiac6 and epicardial electrograms,7 monophasic action potentials,8 and multiple-electrode mapping9 techniques have all been used in a variety of species. However, if the ultimate goal is to understand human physiology and pathophysiology, then the choice of species for experimentation becomes a significant issue because there are considerable interspecies differences. In the case of infarct models, dogs and guinea pigs both manifest extensive coronary collateralization that limits the transmural extent of infarction. The porcine coronary system, however, more closely resembles the human anatomy and therefore serves as a better infarct model.
The murine system deserves special attention, given its increasing use in biologic research. Ease of handling, breeding, and amenability to genetic manipulation has allowed the mouse to supplant many larger animals that were used in classic studies of cardiac electrophysiology. Despite these advantages, the human and the murine cardiovascular systems have clear differences. The average lifespan of inbred mouse strains is approximately 2.7 years, making chronic diseases such as atherosclerosis nonexistent in unmanipulated animals. The significant differences in the coronary anatomy between mice and humans result in different infarct distributions after coronary ligation.10 The average heart rate of a mouse is between 400 and 600 beats/min at rest compared with humans, which is between 60 and 100 beats/min. Murine cardiomyocytes have relatively short action potential durations, making comparisons of the mechanisms of arrythmogenesis and effects of antiarrhythmic drugs with those of their human counterparts difficult (see Figure 1-1, A and B).1,2 These factors must be considered when designing experiments and drawing conclusions from murine studies.
In addition to species-specific limitations, live animal models are confounded by extracardiac variables, for example, neurohormonal effectors that cannot be completely controlled. Live animals are incompatible with the study of unstable ventricular tachyarrhythmias and fibrillation. The type of anesthetic used can significantly alter cardiac electrophysiology. For example, sodium pentobarbital has been shown to alter action potential durations and transmural repolarization gradients by affecting late sodium currents in vivo.11
The Langendorff Heart System
The isolated, perfused heart preparation first described by Oscar Langendorff in 1895 allows physiologic evaluation of the beating heart removed from the neurohormonal and hemodynamic environment of the intact animal.12 The Langendorff heart system consists of an excised heart that is perfused in a retrograde manner by cannulation of the aorta. When optimally perfused, the isolated heart can continue to beat for several hours, allowing detailed analysis of physiologic and pathophysiologic states (Figure 1-3). The advantage of the Langendorff system is the ability to manipulate many aspects of cardiac physiology in a controlled setting. A variety of drugs, toxins, and neurohormonal agents can be infused at specified levels. The isolated heart also provides an opportunity to study arrhythmias that would be hemodynamically unstable in a live animal model. Inducible arrhythmias can be mapped by using standard electrode techniques or optical mapping systems.13 In addition, such modifications as whole-heart preparation allow hemodynamic measurements such as preload and afterload.12
The quality of preparation of the isolated heart is highly dependent on the experience of the investigator. The method of sacrifice before organ procurement and the ischemic time (time interval between heart extraction and initiation of artificial perfusion) can have a significant impact on the quality and internal consistency of the data acquired. Another limitation is that the intact heart makes further delineation of regional myocardial depolarization and repolarization characteristics challenging.6
Wedge Preparations
The ventricular myocardium is a heterogeneous structure consisting of three layers: (1) the epicardium, (2) the mid-myocardium, and (3) the endocardium. The arterially perfused wedge preparation was developed by Yan and Antzelevitch in 1996 to study the electrophysiologic heterogeneity of these myocardial layers in dogs.14 The myocardial wedge is prepared by dissecting along the vascular supply such that the tissue can be perfused during experimentation. By isolating a three-dimensional wedge of ventricular myocardium, the transmural preparation can be studied in electrical and hemodynamic isolation (Figure 1-4).15,16 As with Langendorff hearts, the quality of the wedge preparation depends on the ischemic time. In addition, extensive dissection is necessary to isolate a perfused wedge, leaving significant injury at exposed edges. Wedges that are not adequately perfused must be discarded. Wedge preparations have a limited lifespan, typically 4 to 5 hours, so experiments should be completed within this timeframe.15
Tissue Strips and Slices
Tissue slices are thin preparations of the myocardium that allow diffusion of nutrients and gas exchange. These thin sections can be isolated from various cardiac regions or transmural depths.17 Newer techniques use microtomes to section short-axis slices of whole ventricles measuring 150 µm in thickness.18 Once isolated, activation mapping and action potential characteristics can be evaluated (Figure 1-5).9 Limitations are similar to those of wedge preparations and include the need for long equilibration times (up to 6 hours for epicardial strips) to resolve injury currents before data acquisition.17
Dissociated Myocytes
Dissociated myocytes allow detailed electrophysiologic study of a single cell. Myocyte isolates can be prepared from any species at any developmental stage and from any cardiac region. Isolated myocytes are usually prepared by enzymatic dissociation by using trypsin, collagenase, or a combination of proteases. The ability to study the action potential characteristics of a single cell by using microelectrode techniques has yielded unparalleled insights into how different cell populations functionally contribute to the beating heart. Detailed study of the ion channels that dictate the shape and duration of the action potential could not have been possible without the isolated myocyte. Dissociated myocytes that are virally transformed or harvested from genetically modified mice expressing mutated ion channels have significantly increased the understanding of human channelopathies.19,20 A significant disadvantage of using isolated cells is the loss of the syncytium. Significant changes or exaggeration of action potential features make extrapolations to the intact heart challenging. Furthermore, with prolonged time in culture, the electrophysiologic properties of the myocyte tend to drift and become less representative of the in vivo state.21,22
Artificial Bilayers
Bilayers, unlike an intact cell or native cell membrane, allow the study of ion channels in a more controlled environment. Conditions such as ionic concentration and lipid composition can be precisely manipulated. The artificial bilayer also allows ion channels located on intracellular membranes, such as the ryanodine receptor, to be studied electrophysiologically.23 Single-channel recordings have been performed on numerous channel proteins by using planar bilayer techniques. The two major limitations of this technique are (1) difficulty incorporating ion channels into artificial membranes and (2) the inherent fragility of artificial bilayers.24
Electrode-Based Tools
Extracellular Recordings
Extracellular recordings can be acquired by using either unipolar or bipolar lead systems. The surface ECG uses both bipolar and unipolar recordings. Leads I, II, and III are bipolar leads, and the remaining leads are unipolar. Unipolar leads have a single positive pole and an “indifferent” pole acting as the ground, whereas bipolar leads consist of a positive pole and a negative pole. Unipolar electrograms reflect local and distal depolarization and repolarization events in the heart because they transduce any biopotential above or below the indifferent pole. Bipolar electrograms record local electrical activity by summing the biopotentials recorded by the two poles. The advantages of unipolar recordings are that they provide a more sensitive assessment of local activation; however, inclusion of far-field signals results in a low signal/noise ratio, thus limiting spatial resolution.25 Therefore bipolar recordings are used for detecting discrete electrical events, such as His bundle recordings.26
The electrophysiology study (EPS) uses a combination of unipolar and bipolar electrodes to evaluate the conduction properties of normal and diseased tissue and to perform activation mapping of arrhythmias. In 1996, Charles Berul reported the first in vivo murine cardiac EPS using a combined endocardial and epicardial approach.7 His seminal work described normal sinus node recovery times; atrioventricular (AV) conduction properties; and atrial, AV, and ventricular effective refractory periods in mice. Subsequently, an intracardiac, octapolar electrode catheter was developed to study the murine AV nodal and infra-Hisian conduction system.27 Intracardiac electrograms have been a powerful tool in evaluating transgenic and knockout murine models for conduction disturbances. For example, loss of connexin40 (Cx40), predominantly expressed in the atria and His-Purkinje system, results in significant PR prolongation on surface ECG. Further analysis with intracardiac EPS demonstrated prolonged atrial-His (AH) and His-ventricular (HV) conduction times (Figure 1-6).6,9
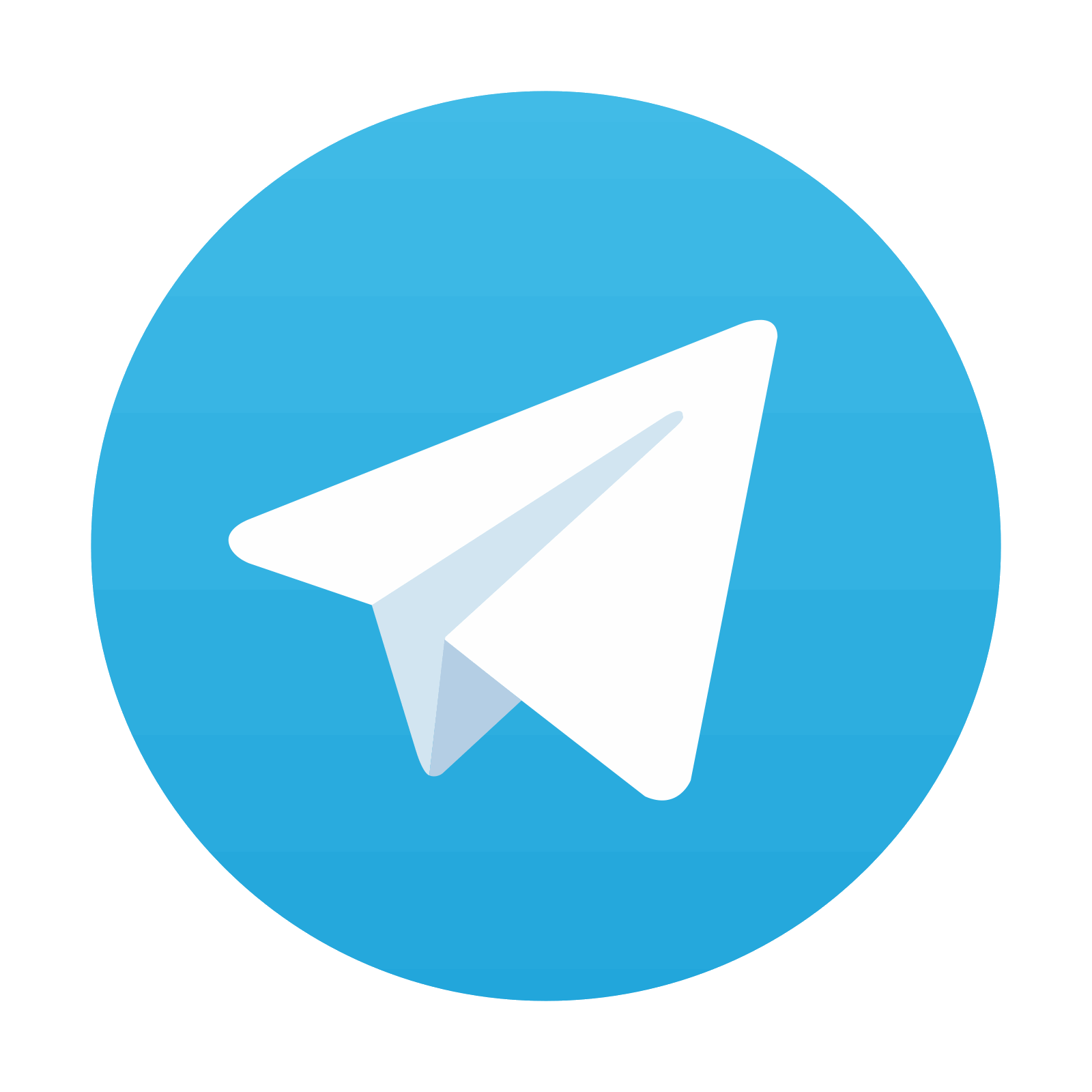
Stay updated, free articles. Join our Telegram channel
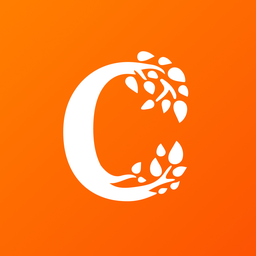
Full access? Get Clinical Tree
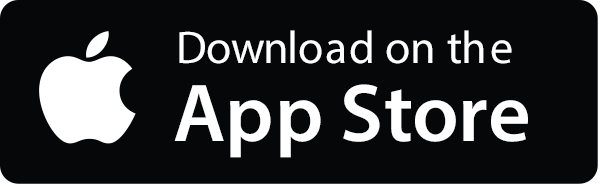
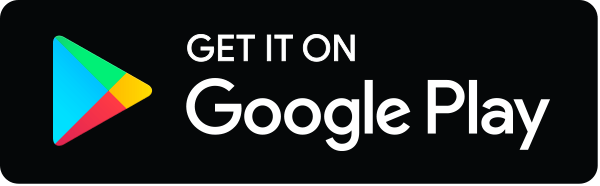