Fig. 2.1.
Saprotroph to pathogen: a three-step cause-and-effect framework. Diseases caused by A. fumigatus arise as a result of both pathogen and host activities, and the transition from saprotroph to pathogen can be considered as occurring in three stages: primary encounter, persistence and permanence. A quantitative understanding of the contributions made by each factor is currently lacking; however, factors that can alter the outcome of the host–pathogen interaction, at the level of the whole animal host, are indicated in italicised, underlined text
III. Primary Encounter
A. Spore Size, Abundance and Human Exposure
A. fumigatus spores are a common component of the airborne microflora and are usually more abundant in outdoor, rather than indoor, air samples (Torpy et al. 2012). Despite seasonal variations in spore abundance, the estimated number of viable A. fumigatus colony forming units per square metre (CFU/m 2 ) rarely exceeds 100, so A. fumigatus spores are certainly not the most abundant of those we normally breathe. Aspergillus spp. are, however, the fungi most often cultured from the lungs of chronically ill humans (Lass-Florl et al. 1999) and since Cladosporium spores greatly outnumber those of A. fumigatus in outdoor air (O’Gorman and Fuller 2008) the spectrum of disease-causing fungi is not reflected by the results of air surveys. Clearly therefore, some mould spores enjoy a positive advantage in the host.
A. fumigatus sporulates abundantly, producing green pigmented, uninucleate, mitotic spores of approximately 2 μm diameter extruded as chains from aerial hyphae positioned high above the vegetative mycelial mass (Fig. 2.2a). This organisation of newly generated spores ensures efficient dispersal of spores in air currents, wherein A. fumigatus spores remain buoyant and viable for long periods of time. Spore dispersal is influenced by air mass movement, turbulence and thermal convection, with physical characteristics such as size, shape, density and surface texture exacting comparatively minor effects. Terminal velocity (or fall speed) is the dominant physical property, and actinomycete spores (approximating 2 μm diameter) are estimated to fall very slowly at approximately 40 μm/s (Lacey and West 2007). According to the scheme proposed by Lacey and West (2007), their extremely small size promotes the deepest possible penetration of the respiratory tree (Fig. 2.2b).
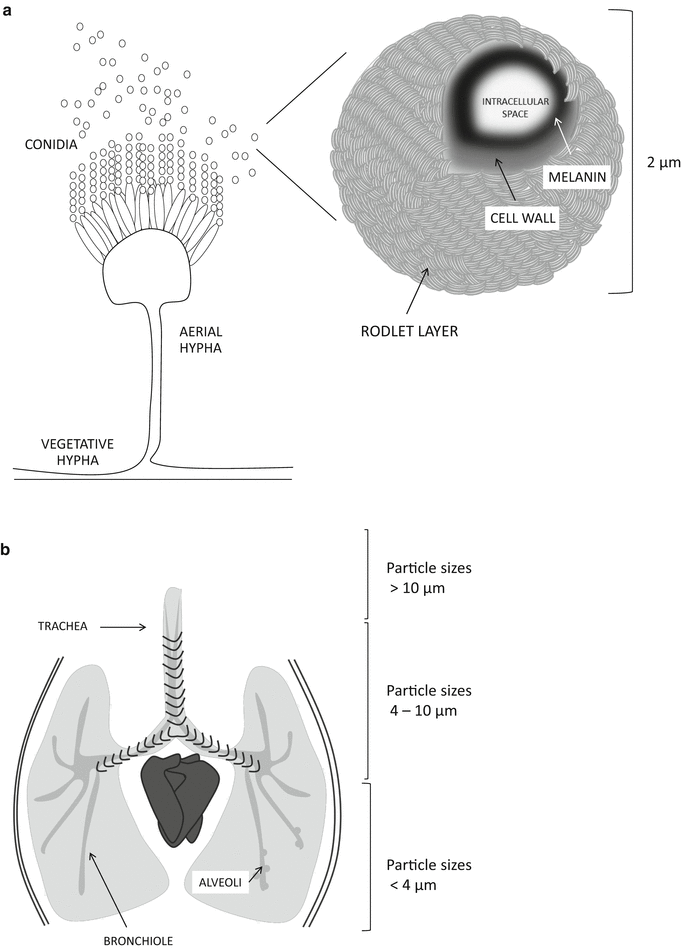
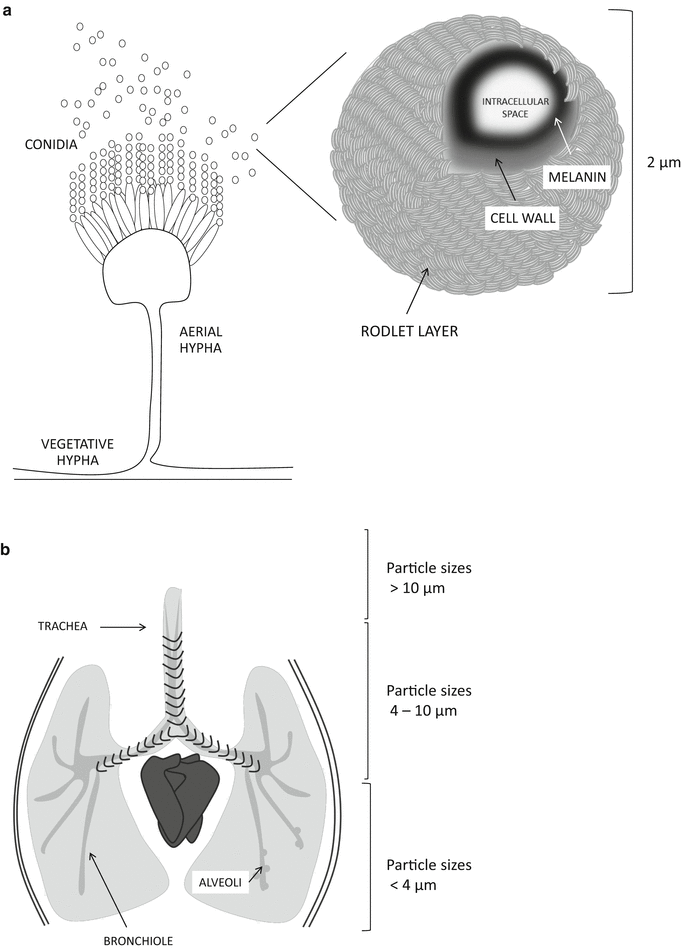
Fig. 2.2.
Organisation of A. fumigatus colonies, conidial structure and airway penetration by particle size. (a) A. fumigatus is a prolific spore producer, generating thousands of uninucleate mitotic spores (conidia) per aerial hypha. Mitotic spores, approximately 2 μm in size, are extruded from aerial hyphae and become airborne. (b) A. fumigatus spores are small enough to achieve deepest possible penetration of the human airway
Stored products, including hay, straw, grain, wood chips and composts, represent sources of A. fumigatus spores that can be aerosolised by human activities. Incredibly, the immediate vicinity of combine harvesters has been found to contain 200 million fungal spores per cubic metre, a concentration capable of eliciting an immediate hypersensitivity reaction (O’Gorman 2011). The potential for exposure to airborne A. fumigatus spores is heightened in the immediate vicinity of refuse dumps and landfill sites, and associated hazards to respiratory health have been formally assessed by Swan et al. (2013). In a recent review of the risk posed by A. fumigatus spores O’Gorman (2011) cites guidelines issued by the UK Environment Agency in 2009 that state that total emissions of bacterial and fungal spores from compost facilities not exceeding 103 CFU/m3 are within “acceptable levels”. Potential environmental reservoirs governing everyday exposures include dust, bedding, water supplies, air handling systems, fresh and dried flowers, the soil of ornamental plants, certain foods including tea, biscuits, fruits and spices, particularly pepper, and the smoking of tobacco and marijuana. Activities such as grass cutting, digging, potting plants, building works and household cleaning also aerosolise A. fumigatus conidia (O’Gorman 2011).
Although initiation of hypersensitivity reactions can be elicited by massive exposures to A. fumigatus spores, infections of immunocompromised hosts are probably initiated by single or repeated exposures to far fewer fungal spores. The true relationship between Aspergillus concentration in the air and the probability of invasive aspergillosis is not quantitatively known, and the circumstances surrounding initiation of infection pose important unanswered questions. It is not certain whether prior carriage of, or repeated exposure to, A. fumigatus spores primes the host for subsequent infection. Some have surmised that large inocula of fungal spores may overwhelm an otherwise adequately functioning immune system (Hope et al. 2005) whereas others have sought correlations between fungal colonisation and subsequent invasive aspergilloses (IA) (Einsele et al. 1998).
Leleu and co-workers (Leleu et al. 2013) used a combination of Bayesian modelling and in vivo experiments to determine the relationship between spore exposure and occurrence of IA. This study determined that in immunosuppressed (neutropenic) mice, exposed by aerosol to A. fumigatus spores, the median exposure dose required to achieve fatal infection of 50% of mice is 1.8–3.2 × 104 A. fumigatus spores. Animals exposed to lower doses were unlikely to contract infections causing death within 6 days of spore exposure. The authors acknowledge that a single exposure to ~ 104 spores is unlikely to occur in humans, where a cumulative dose over time is more likely. However, for patients with prolonged neutropenia or undergoing other immunosuppressive therapies, a significant cumulative infective exposure could be reached within a few hours or days. This study established, for the first time, a direct correlation between aerosol spore burdens and the probability of mammalian infection; however, the relevance of these data to human disease remains speculative because of the marked differences in the exposure conditions and in the respiratory system of mice and humans.
B. Physiological Traits That Favour Pathogenicity
The primary route of infection with Aspergillus is via the inhalation of airborne conidia, which are deposited in the bronchioles or alveolar spaces (Fig. 2.2b). The average size of A. fumigatus conidia (2–3 μm) is ideal for infiltrating deep into the alveoli, whereas larger particles, including the conidia of human pathogens such as A. flavus and A. niger, could be removed more easily by mucociliary clearance of the upper respiratory tract. Evaluation of parameters influencing the germination of Aspergillus conidia revealed significant differences in germination between differing pathogenic species, whereby A. fumigatus germinates much more efficiently and in a shorter time frame than Aspergillus flavus or Aspergillus niger (Araujo and Rodrigues 2004). Following 12 h of incubation in a nutritive medium, the average germination rate of A. niger strains was about 36.5%. A similar rate was obtained more quickly in A. flavus (8 h) and A. fumigatus (5.5 h). At ambient temperatures of up to 30°C, germination rates were similar for all three species; however, at higher temperatures germination of A. flavus decreased by 45%, and in the case of A. niger no germination was possible for 24 h. Thus, among pathogenic Aspergilli, A. fumigatus appears better equipped for initiating growth in the mammalian host.
The importance of thermotolerance for colonisation and infection of mammals is supported by the attenuation of virulence in a mutant lacking the cgrA gene product, which is involved in ribosome biogenesis. ΔcgrA mutants grow normally at 25°C and are fully virulent in a Drosophila insect model (25°C) of infection, but grow slower than the wild-type progenitor isolate at 37°C and are attenuated for virulence in mice (Bhabhra et al. 2004).
C. Host Susceptibility: How Does Immunity Fail the Host?
The potency of A. fumigatus as a pathogen and source of antigens is evidenced by the spectrum of diseases it causes in humans (Fig. 2.3). For example, in severely immunocompromised hosts inhaled spores remain largely unchallenged by innate immune defences and tissue-invasive disease predominates. However, in atopic individuals an over-exuberant response to spore and/or hyphal antigens can prompt life-threatening allergy or chronic pulmonary inflammation (Zmeili and Soubani 2007). Thus, the problem posed by A. fumigatus extends beyond the mere ability to proliferate within the host environment. The disparate outcomes resulting from spore inhalation in immunologically distinct host settings demonstrate the crucial contribution made by the host environment to progression of disease. In humans, Aspergillosis can be broadly grouped into three major categories: invasive aspergillosis (IA; with or without angioinvasion), chronic pulmonary aspergillosis (CPA; including aspergilloma) and allergic bronchopulmonary aspergillosis (ABPA; Fig. 2.3).
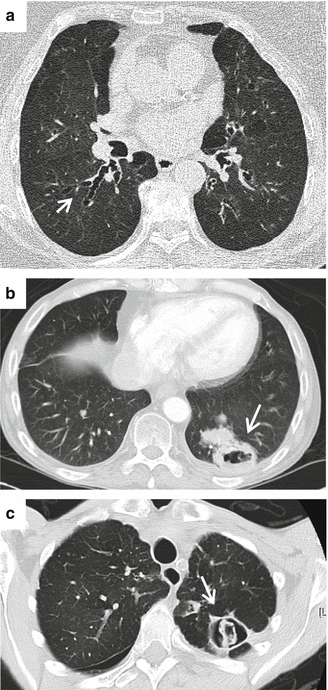
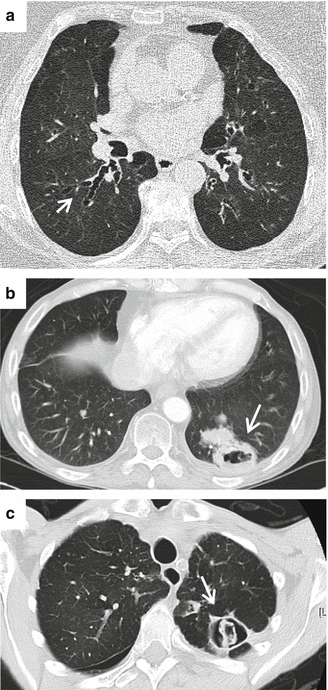
Fig. 2.3.
Outcomes of A. fumigatus spore inhalation in humans. (a) Allergic bronchopulmonary aspergillosis (ABPA). Patient has longstanding ABPA with central bronchiectasis (white arrow). Total IgE is 500 times higher than normal at 5,000 versus 100 IU/ml. Patient has undergone itraconazole therapy for 7 years with stability and no exacerbations (b) Invasive pulmonary aspergillosis (IPA). Heart transplant recipient with a recent episode of rejection requiring pulse corticosteroids in high dose, presenting with chest complaints and headache. A cavitary lesion is apparent (white arrow), and patient also had multiple abscesses in the brain (c) Chronic pulmonary aspergillosis (CPA). A 65-year-old patient with rheumatoid arthritis and rheumatoid lung disease who gradually deteriorated, with weight loss and severe breathlessness over severalmonths. A cavity is evident (white arrow) that shows early signs of aspergilloma (fungus ball)
D. Invasive Aspergillosis
In an authoritative review of the clinical presentations, risk factors and diagnosis of invasive aspergillosis, Hope and Denning (Hope et al. 2005) described an entire spectrum of distinct IA syndromes having differing pathophysiological profiles. For simplicity we here consider IA to include aspergillosis of both the angioinvasive and non-angioinvasive types, among which invasive pulmonary aspergillosis (IPA) is the commonest manifestation. Zmeili and Soubani (2007) defined seven major risk factors for IA: (i) prolonged neutropenia or neutrophil dysfunction (e.g., as seen in chronic granulomatous disease, CGD), (ii) organ or stem cell transplantation (highest risk is with lung and haematopoietic stem cell transplantation, HSCT), (iii) prolonged (>3 weeks) and high-dose corticosteroid therapy, (iv) haematological malignancy (risk is higher with leukaemia), (v) chemotherapy and (vi) advanced AIDS.
In humans, profound neutropenia (< 100 neutrophils/μL) lasting more than 10–15 days is the major risk factor for invasive mould infections (Portugal et al. 2009) and this provides the major basis for assuming that neutrophils provide the most important defence to the human host. Hope and Denning (Hope et al. 2005) distinguish between angioinvasive and non-angioinvasive aspergilloses on the basis of both clinical presentation, and predisposing risk factors whereby angioinvasive disease occurs exclusively in the neutropenic setting and is characterised by vascular invasion by hyphal elements, coagulative necrosis and haemorrhagic infarction. In contrast, non-angioinvasive disease lacks any evidence of vascular invasion but is characterised by any, all or some of: pyogranulomatous inflammatory infiltrate, inflammatory necrosis or cavitation and occurs in multiple non-neutropenic clinical settings, most significantly following corticosteroid therapy, non-neutropenic HSCT, graft-versus-host disease (GVHD), HIV/AIDS, CGD and solid organ transplantation. Murine models approximating the progression of angioinvasive, non-angioinvasive, CGD bone marrow and solid organ transplantation have provided a useful proxy for the study of the host–pathogen interaction as in all instances the major features of disease progression can be replicated (Clemons and Stevens 2005; Lewis and Wiederhold 2005; Balloy et al. 2005; Pollock et al. 1995). The major drawback of murine experimentation is the large infectious inocula that are routinely employed to achieve measurable read-outs of disease progression. Future advances in bioimaging and mathematical modelling will permit the detailed study of diseases resulting from lower inocula.
E. Chronic Pulmonary Aspergillosis
Chronic forms of pulmonary aspergillosis are distinguishable from IA by the timescale of disease progression (Hope et al. 2005). In contrast to IPA, CPAs run a slowly progressive course over weeks to months and include several syndromes, variously characterised by slowly progressive cavitary lung disease, chronic respiratory symptoms and the presence of precipitating antibodies to Aspergillus spp. Hope and Denning (Hope et al. 2005) noted that chronic necrotising pulmonary aspergillosis (CPNA) may present with direct invasion of pulmonary parenchyma by hyphal elements but for the most part no clear evidence of parenchymal invasion can be secured, despite progressive tissue damage. Prior structural lung disease appears to be a crucial factor and defects in systemic immunity may also be important. The precise mechanism of new cavity formation remains unclear, and where cavities are present they may or may not contain fungus balls. The presence, or not, of an aspergilloma provides the basis upon which the distinction between aspergilloma (fungus ball) and CPA (cavitating disease) is made. Aspergillus tracheobronchitis is a further variant of CPA, occurring frequently in solid organ transplantations that incorporate calcineurin inhibition regimens. Tracheobronchitis occurs higher in the respiratory tree and involves profuse, but superficial inflammation with a mucus exudate. The depth of infection ranges from no, to extensive, involvement of the bronchial wall (Herbst et al. 2013; Zmeili and Soubani 2007).
F. Allergic Bronchopulmonary Aspergillosis
Allergic bronchopulmonary aspergillosis (ABPA) is an immunological disorder caused by hypersensitivity to Aspergillus fumigatus and occurs predominantly in people with asthma or cystic fibrosis (Patterson and Strek 2010; Zmeili and Soubani 2007). Sensitisation to Aspergillus prompts activation of T helper 2 lymphocytes, which play a key role in recruiting eosinophils and other inflammatory mediators. Clinical presentations therefore include granulomatous inflammation consisting of histiocytes, lymphocytes and eosinophils. Local inflammation results in mucus production, airway hyperreactivity and, ultimately, bronchiectasis in which fungal hyphae may be seen, but without evidence of tissue invasion. Early diagnosis and treatment is likely to prevent disease progression, parenchymal damage and loss of lung function. To this end, ABPA is defined by a combination of clinical and laboratory criteria that include asthma, serum eosinophilia, elevated total IgE, pulmonary opacity, bronchiectasis and sensitisation to A. fumigatus antigens by skin testing. Treatment with corticosteroids can be effective but may be required indefinitely. The incidence of ABPA in patients with asthma is approximately 2%, and 1–15% of cystic fibrosis sufferers develop ABPA (Sorci et al. 2011; Agarwal 2009; Eaton et al. 2000).
G. Aspergillosis in Animals
Aspergillosis is relatively uncommon in other mammals, but dogs, horses, cows and dolphins are susceptible and birds are particularly susceptible to infection. Avian aspergillosis affects both immunocompetent and immunosuppressed birds, in particular turkeys, penguins, raptors and waterfowl. Immunosuppression caused by forced production or physical exertion can predispose to infection. As in humans, A. fumigatus is the major pathogen (Tell 2005).
H. Spore Composition
A. fumigatus spores are the particles with highest relevance to the primary encounter between host and pathogen. Dependent upon the immunosuppressive regimen adopted, germination of A. fumigatus spores in murine airways can take as little as 6–8 h (severely neutropenic hosts) or more than 24 h (corticosteroid-treated hosts) (Balloy et al. 2005). Spores can therefore enter into extended interactions with host cells prior to germination or elimination. This is a key consideration in the study of the Aspergillus host–pathogen interaction because (a) the antigenic identity of spores, swollen conidia and hyphae differs considerably and (b) in contrast to Candida albicans, which conditionally undergoes filamentation in response to relevant stimuli, A. fumigatus is obliged to undergo the morphogenetic transition from uninucleate single cells to multinucleate hyphae during vegetative growth. During IA, this transition might be rapid or slow, but it will always occur.
Concordant with a role for adhesion to host cells during initiation of mammalian infection, histological analysis of murine lung tissue at early time-points of experimental murine aspergillosis reveals the apparent adherence of spores to alveolar and bronchial epithelial cells. The molecular basis for such adhesion has been probed via in vitro experimentation, revealing that spores of A. fumigatus specifically bind to extracellular matrix (ECM) components of A549 epithelial cells. They also bind specifically to fibrinogen, fibronectin, laminin, type I collagen, and type IV collagen. Pre-incubation of spores with an Arg-Gly-Asp tripeptide inhibits binding to fibronectin and type I collagen by 50% (Tronchin et al. 1997; Bouchara et al. 1997; Penalver et al. 1996; Gil et al. 1996). Both carbohydrate and protein molecules on the conidial surface are involved in binding to host proteins (Sheppard 2011).
The outermost cell-wall layer of the A. fumigatus conidium is adorned with a mesh of interwoven rodlet proteins (Fig. 2.2a), including an immunoprotective rodlet protein RodA, which confers conidial hydrophobicity. A rodlet-lacking A. fumigatus mutant, ΔrodA, (Thau et al. 1994) is defective in adherence to collagen and albumin, but retains the capacity to bind host cells (both in vivo and in vitro) and is fully virulent. In a modified murine model of pulmonary aspergillosis, using milder immunosuppressive drug regimens, Shibuya and colleagues (Shibuya et al. 1999) studied the inflammatory response to infections with A. fumigatus wild-type and ΔrodA conidia. In comparison to mice infected with the wild-type isolate, pulmonary lesions induced by the rodletless mutant were limited and inflammatory responses were weak. The mechanistic basis of this pathogenic phenotype was proposed as being due to RodA-mediated depletion of neutrophils and macrophages during the early stages of infection. However, although this hypothesis has not formally been disproved in whole animal studies, it is now clear that the RodA hydrophobin acts by exerting an immunoprotective barrier to recognition by host cells (Aimanianda et al. 2009).
Early observations of the outermost conidial surfaces, conducted via electron microscopy, determined that spore swelling and germination lead to alterations in surface characteristics and adherence of A. fumigatus conidia. Furthermore, the cell wall was noted to undergo reorganisation during swelling and germination (Tronchin et al. 1995). The ultrastructural appearance of the conidial cell wall differs significantly from that of hyphal cells (Bernard and Latge 2001) whereby the conidial cell wall is composed of a dense pigmented outer layer (containing melanin) and a translucent inner layer (Fig. 2.2a). Wasylnka and colleagues (Wasylnka et al. 2001) showed that A. fumigatus conidia bind significantly better than those of other Aspergillus species to intact lung cell basal lamina and, seeking the mechanism of this binding, found that neither desialylation nor complete deglycosylation of fibronectin decreased the binding of A. fumigatus conidia. This suggested that fibronectin binding was not oligosaccharide-mediated, a finding that was further supported by binding of A. fumigatus spores to a nonglycosylated 40 kDa fragment of fibronectin containing the glycosaminoglycan (GAG)-binding domain. Because binding could be inhibited by negatively charged carbohydrates, the authors proposed a novel mechanism of conidial attachment whereby adherence to fibronectin (and other basal lamina proteins) might be mediated via negatively charged carbohydrates on the conidial surface. In another study, the same authors demonstrated that surface-localised sialic acids, at an estimated density of 7 × 105 sialic acid residues per conidium, mediated adhesion of conidia to the positively charged polymer poly(l-lysine) (Wasylnka and Moore 2000). Thus, sialic acids on the conidial wall may be involved in adhesion to fibronectin as such binding is strongly inhibited in the presence of a sialylated glycoprotein. Sialic acids are terminal components of many glycoproteins and glycolipids, contributing to the structural properties of these molecules and regulating cellular and molecular interactions (Kelm and Schauer 1997). Sialic acid residues can act as, or to mask, recognition sites such as subterminal carbohydrate structures or proteins. Although the role of such residues in mediating the binding of pathogens to host cells has been documented over many years, the identity of the A. fumigatus sialic acid-presenting moiety is unknown.
Levdansky and colleagues (Sharon et al. 2011) performed a molecular genetic analysis of conidial cell wall integrity, initially focusing upon the cell wall-associated protein CspA, originally identified as one of four putatively GPI-anchored cell wall proteins whose gene sequences contained different numbers of DNA repeats in different patient isolates. The predicted cpaA translation product includes a long serine-threonine-proline-rich N-terminal region, followed by a variably (18–47 repeats) large six-amino-acid serine-proline [P-G-Q-P-S-(A/V)]-rich tandem repeat region having significant homology to the repeat domains found in mammalian type XXI collagen. A myc-tagged cspA-encoded protein was detectable in hydrofluoric acid extracts of conidial cell walls, suggesting covalent linkage of CspA to cell wall glucan via a GPI anchor. Further, discrepancy between predicted and actual sizes of the protein suggests heavy glycosylation. Immunofluorescence microscopy revealed a hyphal-specific expression profile (6–12 h post-germination). However, western blotting revealed an abundance of the protein in dormant and swollen conidia. Taken together, these observations suggest that CspA is located below the cell wall surface and becomes unmasked as germination proceeds. In support of this theory, sonication of conidia, intended to remove the outer hydrophobin layer, significantly heightened surface exposure of CspA. In combination with deletions of genes encoding the GPI-anchored cell wall proteins ecm33 or gel2, cpsA deletion additively affects conidal adhesion to culture-derived ECM, and leads to morphological abnormality of the conidial cell wall, which exhibits defective layering of melanin-rich and carbohydrate-rich domains, and high levels of exposed chitin, mannose and glucan. Although these structural reorganisations of the cell wall could be correlated with increased internalisation of the double mutants by human monocyte-derived macrophages, and heightened susceptibility to hyphal damage mediated by polymorphonuclear monocytes, virulence was unaltered in neutropenic mice.
I. Immunological Tolerance of Inhaled Aspergillus fumigatus Spores
Hyperinflammatory responses to inhaled spores occur in patients and mice suffering from CGD (Song et al. 2011; Patterson and Strek 2010) or ABPA (Patterson and Strek 2010). Clearly then, A. fumigatus spores are potently capable of evoking immune responses in the mammalian host and, given the frequency with which A. fumigatus spores enter the human pulmonary tract, mammalian immunity has evolved to cope with this omnipotent challenge. In 2009, Aimanianda and co-workers (Aimanianda et al. 2009), in attempting to dissect the reasons for the immunologically inert nature of the dormant conidia, made some findings that could explain this phenomenon. Analysis of a hydrofluoric acid extract derived from A. fumigatus conidia identified three protein species, the molecular weights of which (32, 16 and 14.5 kDa) corresponded, respectively, to the dimeric form of the native RodA protein, native RodA and partially degraded or processed RodA. Crucially, RodA was undetectable in fungal culture supernatant following conidial germination, indicating that the RodA rodlet layer, covalently bound to the cell wall of dormant conidia (Figs. 2.2 and 2.4), was shed upon spore germination. RodA extracted directly from A. fumigatus condia was found to be immunologically inert. This is in contrast to germinating conidia, which prompt, in dendritic cells, significant expression of co-stimulatory molecules (CD80, CD86, CD40 and CD83) and the antigen-presenting molecule human leukocyte antigen DR (HLA-DR), and secretion of pro- and inflammatory and anti-inflammatory cytokines. The removal of RodA, either chemically (using hydrofluoric acid), genetically (ΔrodA mutant) or biologically (germination) resulted in immune activation. Thus, the hydrophobic rodlet layer on the A. fumigatus conidial cell surface acts as an “immunological silencer”. A similar effect was documented in murine alveolar macrophages where inflammatory cytokines, chemokines and reactive oxygen intermediates (produced in response to hydrofluoric acid-treated conidia, ΔrodA dormant conidia and germinated conidia) were undetectable in macrophages exposed to dormant conidia or RodA protein. The authors concluded that the surface rodlet layer of the conidial cell wall makes A. fumigatus conidia inert to both innate and adaptive immunity. Evidence first documented by Hohl et al. (2005) revealed a further layer of immunomodulatory control, manifested by the gradual exposure of cell surface β-glucan during swelling and germination of A. fumigatus spores (see subsequent discussions).
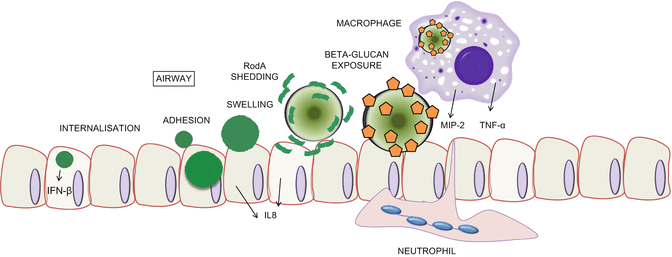
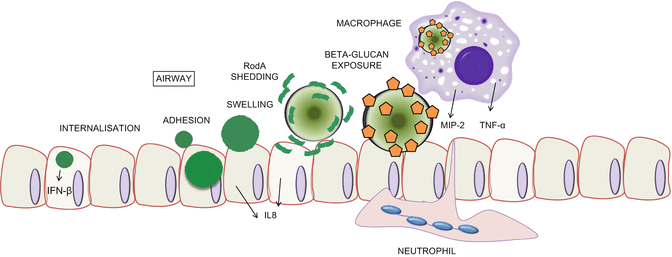
Fig. 2.4.
First encounter, immune evasion and fungal persistence in the host. A. fumigatus spores, both resting and swollen, can prompt inflammatory responses in epithelial cells (ECs). ECs internalise both resting and swollen spores, eliciting differential responses to each morphotype. A. fumigatus spores are adorned with a layer of proteinaceous rodlets, which are shed upon swelling to reveal cell-wall-associated polysaccharides, some of which act as pathogen-associated molecular patterns. Orange pentagons represent β-glucan moieties
IV. Persistence
A. Spore Germination
In environments conducive to germination, A. fumigatus spores become metabolically active and increase in size, eventually germinating to produce tip-extending elongated cells called hyphae, a hallmark characteristic of filamentous fungi. In most fungi, conidial germination is governed by moisture, oxygen and nutrients and, when dormancy is broken, A. fumigatus conidia begin nuclear division and morphological development (Momany and Taylor 2000). This morphological transition multiply impacts upon A. fumigatus pathogenicity (Fig. 2.4), from shedding of the RodA hydrophobic layer (Aimanianda et al. 2009) to gradual exposure of pathogen-associated molecular pattern molecules (PAMPs) and activation of immune responses (Hohl et al. 2005) and invasion of the host epithelium (Kamai et al. 2009).
Recognising that morphological landmarks coincide with specific events in the S. cerevisiae cell cycle, Momany and Taylor (2000) sought similar landmarks in the transition of A. fumigatus and Aspergillus nidulans from conidia to hyphae. To score A. fumigatus cells for mitotic and morphological events, Hoechst 33258 and calcofluor white, respectively, were used to stain fungal cells. Following inoculation into rich medium, the first nuclear division in A. fumigatus occurs after a lag of just over 4 h, with subsequent nuclear divisions every 45 min. This represents a significantly faster rate of nuclear duplication than that observed in A. nidulans, where nuclei divide once per hour. Initially spherical, conidia of both A. fumigatus and A. nidulans assume a pear-type shape that indicates that an axis of polarity has been established. This event often (22%) happened prior to the first nuclear division in A. fumigatus cells, which also differs significantly from the less pathogenic A. nidulans where only 12% were polarised after the first mitosis. A further important difference between the morphogenetic transitions of these two species included a reduction in second germ tube emergence in A. fumigatus (19% versus 98%) after the fifth mitosis. The authors proposed that by directing growth primarily in one direction, the lower percentage of second germ tubes allows A. fumigatus to scavenge nutrients more efficiently in the host.
Dague and colleagues (2008) used real-time atomic force microscopy with a temperature-controlled 37°C stage to probe the structural and Physiochemical dynamics of single A. fumigatus conidia during germination. By capturing images of the same cell after 20, 60 and 120 min, significant swelling could be quantified. Ultrastructural alterations were imaged via high-resolution images of the spore surface (Fig. 2.2a). At early germination times, the spore surface was observed to be covered with an array of 10 nm diameter rodlets. Notably, dramatic changes in cell surface structure were observed after 2 h of germination, corresponding to the swelling of the conidium. At this point the rodlet layer was found to alter into a layer of amorphous material, thereby buttressing the hypothesis that significant remodelling of the cell surface, including shedding of RodA (Aimanianda et al. 2009), represents a significant restructuring event during spore germination.
From a molecular perspective, the regulatory mechanisms that govern conidial germination in A. fumigatus have not been fully described, and much is extrapolated from studies in the model ascomycete A. nidulans. However, the number of gene products that have been demonstrated as important for conidial germination is rapidly growing, and a plethora of functional categorisations have been implicated, from calcineurin-mediated signalling (da Silva Ferreira et al. 2007; Steinbach et al. 2006) to MAPK signalling (Liebmann et al. 2004; Xue et al. 2004; Zhao et al. 2006) and glucose metabolism (Fleck and Brock 2010). An integrated and hierarchical understanding of the process will gradually emerge once functional genomics approaches reveal the entire cohort of gene products involved.
Fortwendel et al. (2006) recently established the mechanism by which a membrane-associated Ras GTPase switch, RasA, governs morphological transitions in A. fumigatus. Ras-GTPases regulate a multiplicity of cellular processes in a highly precise spatial and temporal manner, commanding control over differential pathway activation, which is governed in part by post-translational modification and consequent modulation of subcellular localisation. Deletion of RasA in A. fumigatus causes delayed germination and subsequent formation of wide, blunted hyphae that continually switch polarised growth axes.
A functional RasA-GFP translational fusion was used to interrogate the correlation between RasA localisation and morphogenesis. RasA was found to localise at the cell periphery of emerging germ tubes and the outer edge of hyphae, consistent with a plasma membrane association of the protein during polarised hyphal growth. Incubation of fungal cultures with a nonreversible inhibitor of protein palmitoylation inhibited hyphal growth in a dose-dependent manner and prompted aberrant localisation of GFP-RasA to internal foci. Taken together, these observations suggested that palmitoylation might be important for proper RasA localisation and polarised hyphal growth. The targeted double mutation (RasAC206/207S) of a conserved RasA palmitoylation motif prevented full complementation of the RasA hyphal growth blockade and resulted in a dramatic reduction of GFP signal at the plasma membrane. GFP-RasAC206/207S was mislocalised from the hyphal periphery and septa to the cytosol and internal patches, presumably to compartments of the endomembrane system. Both a RasA null mutant and the GFP-RasAC206/207S mutant displayed low germination rates and formed stunted, highly branched germlings during early phases of hyphal growth and displayed heightened sensitivity to cell wall damaging agents and aberrant deposition of β-glucan. To determine the contribution of plasma membrane localisation of RasA to A. fumigatus virulence, the ΔrasA and GFP-RasAC206/207S mutants were compared to the wild-type strain in a murine model of invasive aspergillosis. Virulence of both the ΔrasA and GFP-RasAC206/207S mutants was significantly attenuated (P < 0.0001), with infected mice displaying 25% and 20% mortality at 15 days postinoculation, respectively. Appropriate RasA localisation to the plasma membrane is therefore essential for full virulence in A. fumigatus.
Several groups have applied functional genomics techniques to the study of the conidial to hyphal transition in A. fumigatus. Lamarre and colleagues (2008) adopted a transcriptomic approach to analyse the exit of A. fumigatus spores from dormancy, identifying a shift from fermentative to respiratory metabolism and immediate protein synthesis upon breaking dormancy. In the first 30 minutes of incubation in YPD medium at 37°C, modulation of expression of 787 genes (of a possible 3,000) was observed in freshly harvested conidia. Downregulated genes encoded functions involved in fermentative metabolism and oxidoreductase activity whereas upregulated genes indicated a heightened emphasis upon RNA and phosphorus metabolism, amino acid and protein biosynthesis and protein complex assembly. Concordant with the predicted timing of mitosis in germinating spores, the authors found no evidence of upregulation of DNA processing/replication or cell cycle regulation. Additionally, expression of multiple genes involved in amino acid biosynthesis were upregulated during the first 30 minutes of the study, perhaps reflecting the rapid depletion of a pre-existing pool of free amino acids. This trend was supported by observed upregulation of genes in other pathways leading to amino acid biosynthesis, including glutamate dehydrogenase and glutamate synthase, NADPH isocitrate and succinate dehydrogenase. Downregulation of 28 mitochondrial genes, along with genes encoding alcohol dehydrogenases, lactate dehydrogenase, pyruvate decarboxylase and phosphoenol pyruvate synthase suggested a shift from a fermentative metabolism when the conidium is in a dormant stage to a respirative metabolism as soon as the germination process has started. Combining microarray and quantitative proteomic data, Cagas et al. (2011) were also able to identify a trend towards protein biosynthesis in time-series analyses of germinating conidia, during 0–16 hours of growth. A further quantitative proteomic analysis performed by Suh et al. (2012) identified an abundance of small, lineage-specific proteins amongst the conidial proteome. Teutschbein and colleagues (2010) applied two-dimensional polyacrylamide gel electrophoresis to establish a reference map of conidial proteins, identifying 449 different proteins, 57 of which were more abundant in conidia relative to mycelia. These included enzymes involved in detoxification of reactive oxygen intermediates, pigment biosynthesis and conidial rodlet layer formation. In agreement with the transcriptional analyses of Lamarre et al. (2008), pyruvate decarboxylase and alcohol dehydrogenase were detectable in dormant conidia, further supporting the notion that alcoholic fermentation plays a role during dormancy or early germination.
These studies set the scene for analysing differences between germination undergone in vitro and germination occurring during mammalian infection. Although the technologies to analyse the infecting fungal proteome thus far elude us, the transcriptional data from murine infections are already under analysis as part of our research programme.
B. Thermotolerance
The ability to thrive at 37°C is characteristic of all human pathogens and distinguishes A. fumigatus from most other environmental moulds. Relative to other Aspergillus spp., A. fumigatus has a natural propensity for thermotolerance, and in being capable of growth at temperatures that approach the upper limit for all eukaryotes, approximates an extremophilic mode of growth in the human host (Bhabhra and Askew 2005; Bhabhra et al. 2004). An intuitive hypothesis is that mutants that fail to grow at 37°C will be attenuated for virulence. This holds true in at least once case as A. fumigatus mutants lacking the cgrA gene product are attenuated for virulence. CgrA is required for the synthesis of ribosomes during conidial germination and has a distinct nucleolar localisation in A. fumigatus.
Mutants deficient in trehalose biosynthesis also demonstrate thermosensitive phenotypes in A. fumigatus (Al-Bader et al. 2010). Contingent with a role in stress tolerance, the trehalose content of A. fumigatus hyphae becomes elevated in response to heat shock and two genes, tpsA and tpsB, whose expression can be correlated with heightened trehalose content, serve partially redundant roles in trehalose accumulation during development and heat shock. A ΔtpsAB double mutant is devoid of trehalose and exhibits delayed (by 2–3 h) germination at 37°C and is nonviable at 50°C. However, virulence of the double mutant was unabated in a murine model of aspergillosis. Trehalose is regarded as an important source of energy during fungal development and acts to heighten stress tolerance by preventing the aggregation of denatured proteins. Being absent from mammalian cells it is of obvious therapeutic interest. It is intriguing that, despite the prevailing belief that rapidity of spore germination at 37°C provides a competitive advantage for A. fumigatus in the face of the host environment (Araujo and Rodrigues 2004), a mutant such as the ΔtpsAB double mutant does not suffer any deficit in the diseased mammalian host. A plausible explanation put forward for this involves aberrancy of cell wall biosynthesis in the double mutant, which might exacerbate the immune response sufficiently to worsen disease outcome.
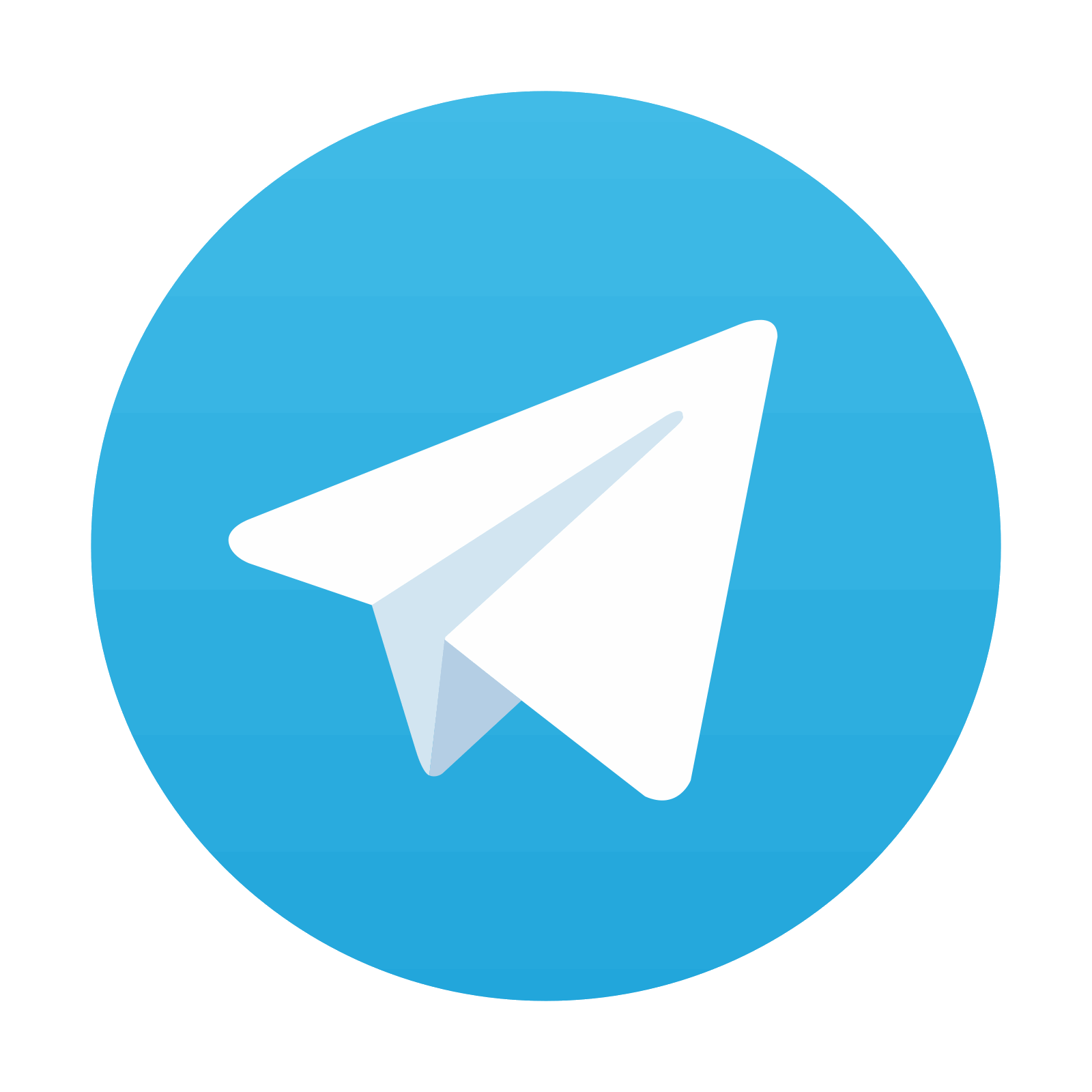
Stay updated, free articles. Join our Telegram channel
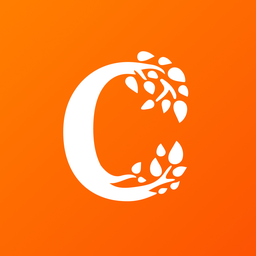
Full access? Get Clinical Tree
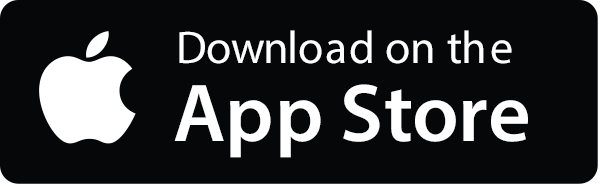
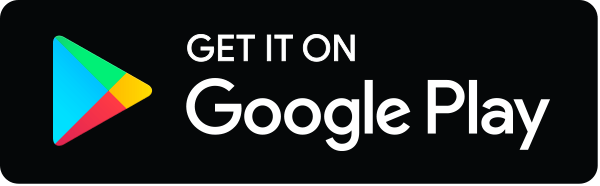