Introduction
The human lung has a large surface area, which for an average-size person approximates half a doubles tennis court, thus maximizing the approximation and apposition of capillaries to the epithelial surface. Whereas this design optimizes gas exchange, it also has the intrinsic risk of exposing the delicate alveolar tissues to potentially noxious particles that may be present in the ambient air. There are various safeguards against the danger posed by inhaled particles. The first line of defense is that the configuration of the nasopharynx and serial branching of the airways causes particles to deposit proximal to the more vulnerable alveolar structures. Second, if an insoluble particle deposits in the lung, there are processes by which it can be cleared from the airways or alveoli. Clearance of insoluble particles that deposit in the ciliated airways is achieved by mucociliary clearance. The particles are trapped in a mucous blanket, which is then transported proximally by beating cilia. If the cilia are not functioning optimally or if the quantity/quality of the airway mucus is abnormal, the mucus and its entrapped particulates can be cleared by cough. Particles that deposit distal to the ciliated airways are removed by alveolar clearance, mainly by macrophages ingesting the particles and transporting them to regional lymph nodes.
The branching structure of the airways is a barrier not only to noxious environmental aerosols but also to therapeutic aerosols. However, the application of the principles of deposition facilitates the design of therapeutic aerosol development. For the most part therapeutic aerosols are “targeted” to the lungs, that is, they are designed to treat lung diseases directly and avoid systemic toxicity. Theoretically, the lungs’ large surface area can facilitate the systemic delivery of nonrespiratory drugs via the lung as aerosols, usually proteins such as insulin that cannot be given by the oral route. However, to date, the use of this pathway has not had a major impact on clinically successful systemic therapeutic drugs.
Definition and Description of an Aerosol
An aerosol can be defined as a system of solid particles or liquid droplets that can remain dispersed in a gas, usually air. Naturally occurring aerosols, as well as those emitted by clinical aerosol generators, almost always contain a wide range of particle sizes. Because the aerodynamic behavior of an aerosolized particle is critically influenced by its mass, it is important to be able to describe precisely the size distribution of aerosolized particles. In clinical studies the mass median aerodynamic diameter (MMAD) and the geometric standard deviation (σg) are often used to characterize the dimensions of an aerosol. When the mass distribution of particles in an aerosol is fractionated and the cumulative particle distribution plotted as a lognormal distribution on probability paper, it often approximates a straight line. However, recent studies of clinical aerosols have indicated that nebulized particles are often not lognormal in distribution. The MMAD represents the point in the distribution above which 50% of the mass resides, expressed as the diameter of a unit density (1 g/mL) sphere having the same terminal settling velocity as the aerosol particle in question, regardless of its shape and density.
The lognormal plot is convenient because, if linear, it defines a statistically “normal” distribution and the data can be described accurately by the MMAD and the standard deviation alone. For a lognormal distribution, one standard deviation is called the “geometric standard deviation,” or σg. The σg is the ratio of the size at 84% (or 16%) to the MMAD and is an indicator of the variability in particle diameters. If the particle size varies over a wide range (σg > 1.2), it is described as having a polydisperse particle distribution; if the particles are of similar size (σg < 1.2), the particle distribution is described as monodisperse. Monodisperse aerosols are usually encountered only in research studies where specialized generators are used to create such an aerosol. For clinical aerosols that are not lognormal and are widely polydisperse, it is best to relate deposition studies to the entire distribution of particles and avoid focusing on simple descriptive terms like the MMAD and σg.
The definition of the mass median diameter is the same as that of the MMAD except that the data are not normalized to unit density.
Principles of Deposition
The fraction of inhaled particles that deposit (as opposed to being exhaled) is called the “deposition fraction.” The likelihood that a particle will deposit in a particular airway depends on the interaction of three factors: the physical characteristics of the particle (e.g., mass, shape), the gas flow in which the particle is transported (the patient’s breathing pattern and any velocity provided to the particle by a propellant), and the airway anatomy (especially the presence of airway obstruction ). In general, the greater the mass, the faster the velocity, and the narrower the airway, the greater the predisposition of the particle to deposit by a process called “inertial impaction.” Inertial impaction is the dominant mechanism by which particles deposit in the nasopharynx and more proximal airways and describes the process by which a particle fails to follow the air stream in which it is suspended, thereby impacting on an obstacle instead of circumventing it. The probability of a particle undergoing inertial impaction (I) can be estimated using the following equation:
I = α ( V t V a sin θ /gR )
Gravitational sedimentation is the process by which a particle accelerates under gravity until it reaches a terminal settling velocity (V t ) , which is determined by the equation
V t = ( ρ − σ ) gd 2 / 18 γ
Sedimentation is critically dependent on the patient’s breathing pattern. If there is a breath-hold before exhalation, particles are more likely to sediment; without a breath-hold, particles are more likely to be exhaled rather than deposited. It has also been suggested that alveolar volume may affect deposition fraction (larger air spaces require more time for sedimentation).
Very small particles (<0.2 µm) can deposit by diffusion. The diffusion coefficient of a particle (D) can be expressed as
D = kT/ 3 π η d
These tiny particles are rarely important in therapeutic aerosols, but in inhalation toxicology studies these particles can be produced by combustion and may be clinically relevant, even though they tend to be transient because of the tendency to agglomeration. In addition, man-made nanoparticles, which are of increasing interest in electronics and biomedical research, would, if inadvertently inhaled, be likely to deposit by diffusion (discussed later under “Environmental Aerosols”).
Particles between 0.2 and 0.5 µm in diameter tend to be too small to deposit efficiently by sedimentation and yet too large to deposit efficiently by Brownian diffusion and tend to be exhaled rather than deposited in the lung.
Measurements of Particle Size
Evaluation of Aerosol Particle Size
Particle size is an important factor in determining whether a particle will undergo nasopharyngeal deposition, airway deposition, or alveolar deposition. Particle size is usually measured by light scattering or cascade impaction: light scattering is based on the principle that there is differential scattering of polarized light by particles of different sizes; cascade impaction is based on a different trajectory of particles of different mass. In cascade impaction, particles at a set flow rate go through a series of apertures of decreasing diameter and impact on a series of plates if they fail to follow the air stream.
Cascade impactors with high flow rates (e.g., 28 L/min) were originally designed for environmental sampling of ambient air to collect 100% of the emitted dose. They have been adopted as the method of choice for monitoring quality control in a manufacturing plant for aerosol delivery. Cascade impaction has an advantage over light scattering in that it facilitates the correlation of different methods of quantifying the distribution of the active drug (e.g., chemical analysis of drug on each plate compared with radioactive label or weight). However, measuring the emitted dose of aerosol using a high-flow cascade impactor is not ideal for predicting how an aerosol will perform in clinical practice. Although low-flow cascade impactors sample only some of the output, they more closely duplicate in vivo conditions.
Application of in Vitro Measurements of Particle Size to Clinical Studies
Particle size measurements using different techniques are not necessarily interchangeable. Meaningful comparisons of the sizes of clinical aerosols, especially those produced by a pressurized metered-dose inhaler (MDI; which emits high-velocity particles), can be made only if obtained with identical techniques. Nevertheless, despite the technical difficulties encountered in measuring the size of polydisperse clinical aerosols, some investigators have established that, when used with appropriate caution, data obtained by in vitro measurement of particle size do provide useful predictive data for subsequent clinical studies.
The classic studies of the influence of particle size on lung deposition were performed with monodisperse aerosols consisting of particles that would not absorb moisture from the air (nonhygroscopic) . In contrast, pharmaceutical aerosols tend to be polydisperse liquid droplets, with the active pharmaceutical ingredient (as well as in some cases pharmaceutically inactive excipients such as preservatives and freon) being either in solution or suspended as micronized particles. Droplets can change size by exchanging water with either the dry carrier gas or the humid environment of the upper airway. In addition, some drug ingredients are hygroscopic, and some diluent solutions are hypertonic, both of which could lead to an increase in particle size; the clinical significance of such changes is not known with certainty. It has been reported that hypotonic saline aerosols were associated with a slightly more peripheral deposition pattern on gamma scintigraphy compared with hypertonic saline aerosols. Measuring the particle distribution of droplet aerosols is difficult because the particles can be affected by ambient humidity. Therefore the predictive value of cascade impaction data from droplet aerosols for in vivo deposition (e.g., for upper airway deposition as measured by gamma scintigraphy) is strongly dependent on the specific technique used for cascade impaction.
Generation of Therapeutic Aerosols
There are three common ways in which therapeutic aerosols can be generated. First, the patient’s inspiratory airflow can aerosolize a micronized dry powder (dry powder inhalers). Second, micronized particles can be suspended in a volatile pressurized liquid that vaporizes when at atmospheric pressure, thus imparting velocity to the emitted particles (MDIs). Third, a dispersal force can be applied to a liquid to generate droplets, which are then inhaled by tidal breathing. The generation of the dispersal force can be as simple as using a compressed air source attached to a narrow orifice to generate a Venturi effect (i.e., jet nebulizer) or by more complex vibrating membranes and meshes.
Dry Powder Devices
Because dry powder inhaler aerosols are generated by the patient’s inspiration, they are by definition coordinated with inspiration ( Fig. 11-1A ). In optimizing the design of new devices, there is a need to minimize resistance to flow so that weak and tachypneic patients will be able to generate a threshold flow rate. Furthermore, it is desirable that the emitted dose should not vary significantly with changes in inspiratory flow so that intersubject dosing remains consistent. For high-potency drugs, lactose is often used as an inert, bulking agent. Devices can be single-dose devices in which a capsule of powder is perforated in the device (Spiriva HandiHaler, Boehringer Ingelheim, Ridgefield, CT), multidisk with individual doses wrapped in foil blisters (Advair/Seretide Diskus, GlaxoSmithKline, Research Triangle Park, NC), or multidose metered reservoir (Pulmicort Flexhaler, AstraZeneca, Wilmington, DE,). For dry powder devices to maintain reproducible dosing over time, it is critical that the powder be protected from moisture. Despite these technical challenges, the design of dry powder inhalers has greatly improved, and the devices described previously perform at high levels of efficiency and reproducibility.

Pressurized Metered-Dose Inhalers
Pressurized MDIs using chlorofluorocarbon propellants have been used in clinical practice for 50 years and remain the most popular method of administering short-acting rescue inhalers and inhaled corticosteroids. They are portable and discreet. The velocity of the emitted particles, however, requires that for adequate lung deposition, there must be precise coordination with a patient’s respiration. Studies have shown that actuation technique is suboptimal in most patients. Even with optimal technique, 80% of the emitted dose may deposit on the pharynx and can cause local irritation (see Fig. 11-1B ). In addition, some orally bioavailable medications cause significant systemic exposure from swallowed medication. Valved holding chambers reduce pharyngeal deposition because high-velocity particles impact on the inside of the chamber and, if the chamber has valves, the need for precise coordination with respiration is obviated. For young children, a face mask can be used in conjunction with a valved holding chamber. Unfortunately, registration studies for MDI medications are almost always performed without a holding chamber because manufacturers do not want their product’s prescription tied to a specific holding chamber. To make the chambers more acceptable to patients, effective small-volume chambers (140 mL) and collapsible chambers have been developed.
For the past decade there has been a phase-out program for chlorofluorocarbon-containing pressurized MDIs because of concerns about the impact of chlorofluorocarbons on the environment. Short-acting β-agonists and corticosteroid pressurized MDIs have been reformulated using hydrofluoroalkane as a propellant. The U.S. Food and Drug Administration mandated that these new hydrofluoroalkane-containing pressurized MDIs have performance standards at least equal to existing chlorofluorocarbon-containing pressurized MDIs. Although this task has been far more difficult and expensive than anticipated, the last remaining chlorofluorocarbon-containing pressurized MDIs were removed from the U.S. market in early 2009.
Jet Nebulizers
The ubiquitous small-volume jet nebulizer is the mainstay of bronchodilator delivery in hospitalized patients and patients at the extremes of age. These devices are cheap and require little patient cooperation. In addition, they are useful for delivering medications that have a relatively large mass such as antibiotics. Generation of the driving pressure requires either a tank of pressurized gas or an electric compressor. Although the manufacturers of compressors have made these devices more portable, they are far from convenient for ambulatory patients. In addition, conventional nebulizers tend to take 10 to 20 minutes to deliver a single treatment, which interferes with adherence. Most generic nebulizers are inefficient and deliver less than 10% of the nebulizer charge to the lung. The remainder is left in the nebulizer chamber as so-called dead volume (droplets and dried particles left on the nebulizer walls), lost through the expiratory port because the device generates aerosol throughout the respiratory cycle, or deposited in the extrapulmonary upper airway because most of the emitted dose is contained in large, poorly respirable particles.
New Developments in Aerosol Delivery Systems
Manufacturers have made enhancements in nebulizers. Internal recycling baffles reduce emitted particle size. Expiratory filters have been added for potentially hazardous aerosols to reduce collateral exposure. Significant enhancements in drug delivery by nebulizers are possible by coordinating nebulization with inspiration (e.g., “breath actuation”) that essentially turns the nebulizer off during expiration. Another improvement in efficiency is called “breath enhancement,” which uses the patient’s inspiratory flow through the nebulizer to increase drug delivery (e.g., LC Star, Pari, Germany; Ventstream, Medicaid, Bognor Regis, UK).
The newer devices include the AKITA (Activaero GmbH, Gemünden, Germany), AER X (Aradigm, Hayward, CA), eFlow (Pari, Midlothian, VA), and I-neb (Philips/Respironics, Pittsburgh, PA). Each device employs unique proprietary technologies that distinguishes it from the traditional devices. Some tend to be more precise in their coordination with breathing patterns of individual patients (AKITA, I-neb, AERx); they usually have a lower dead volume in the nebulizer chamber, a greater fraction of particles in the respirable range (i.e., <5 µm), and shorter treatment times. They eschew bulky compressors, use either vibrating mesh or crystal, or extrude the liquid through tiny holes or a combination of both vibration and microextrusion. They vary in their durability and the relative ease with which they can be cleaned between treatments.
Recently the design of aerosol delivery systems has combined slow and deep inspiration with direct mechanical feedback to the patient for particles of relatively large MMAD. As discussed earlier, a “slow” inspiration reduces particle inertia and allows inhalation of particles that would otherwise deposit in the oropharynx during tidal breathing and will thus minimize oropharyngeal deposition.
Principles of Assessment of Delivery Systems
Assessing effects of an aerosolized drug requires the understanding of three major factors: the characteristics of the aerosol delivery system, the quality of the aerosol produced, and the quantification of deposition within the lungs. Quantification of deposition is performed in vivo and is time consuming and costly and involves some degree of risk and uncertainty to the patient. The other two components of the aerosol delivery process can be well characterized and studied in vitro. The field of aerosol delivery has advanced significantly in the last 10 years so that aerosol delivery characteristics and the quality of the aerosol can be significantly optimized on the bench before exposure to patients.
The Inhaled Mass
Figure 11-2 depicts a simple in vitro setup for measuring the quantity of aerosol produced by a nebulizer. A filter that captures the aerosolized particles has replaced the mouthpiece. This system does not require an understanding of nebulizer function from first principles. Because the nebulizer is attached to a breathing device (Harvard pump, Harvard Apparatus, South Natick, MA), the conditions of delivery such as routine tidal breathing can be duplicated. The quantity of drug captured on the inspiratory filter represents the amount that passes the lips of the patient. To distinguish this quantity from a “dose” or deposited drug, the term inhaled mass has been coined. The inhaled mass represents “delivery” of drug to the patient constrained by conditions that should mimic actual clinical delivery.

Deposition
The term deposition begins to imply a “dose” to the patient. The term deposition needs to be further refined in a given situation (e.g., oropharyngeal versus parenchymal deposition, or central versus peripheral deposition within the lung). Each of these terms may be important depending upon the disease entity to be treated. Obviously, the measurement of the actual deposition requires an in vivo experiment. However, deposition can be estimated based on parameters that are measured in vitro as shown in Equation 4 :
Deposition = inhaled aerosol − exhaled aerosol
Because the term aerosol is a little vague with respect to drug activity, Equation 4 can be rewritten as
Deposition = inhaled mass − exhaled mass
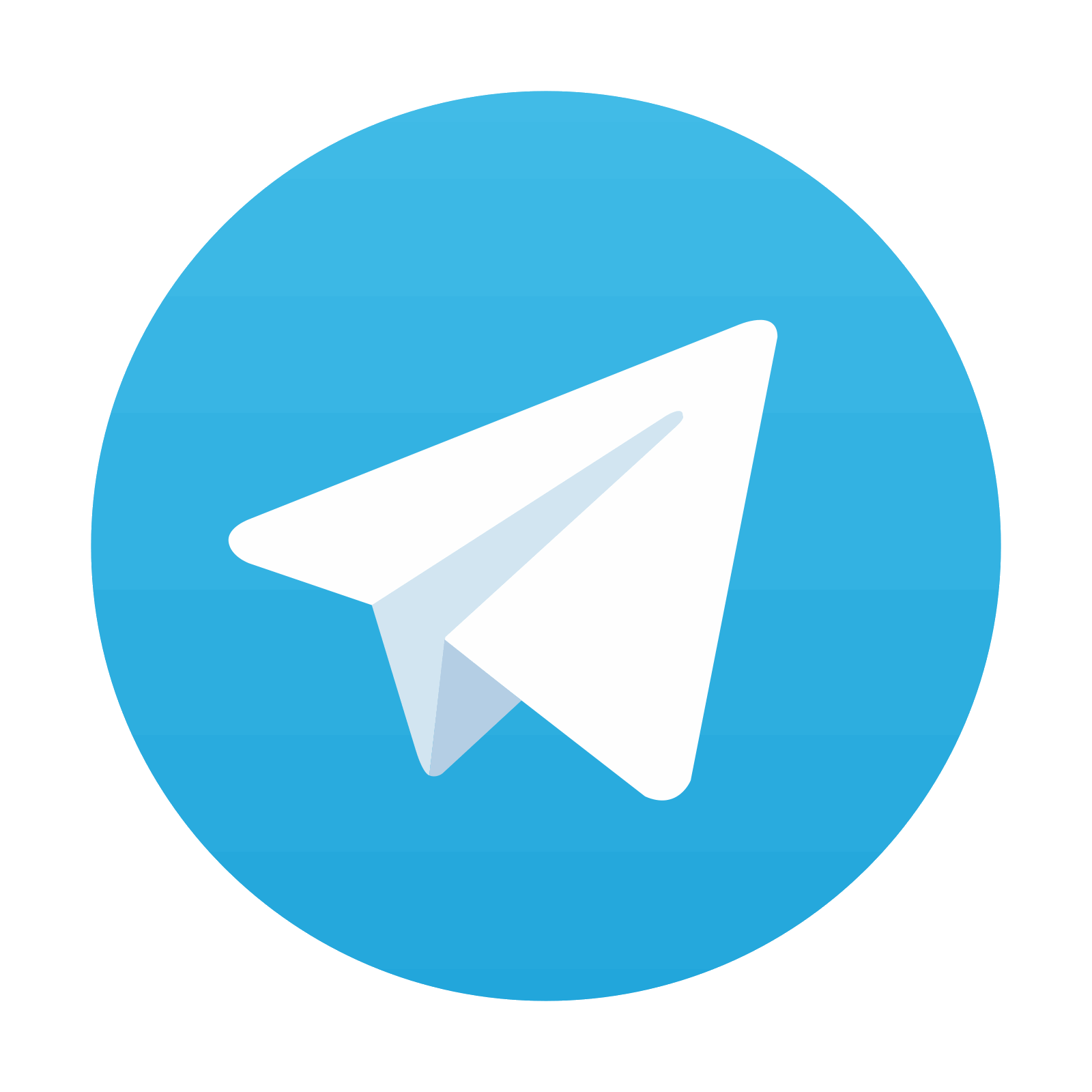
Stay updated, free articles. Join our Telegram channel
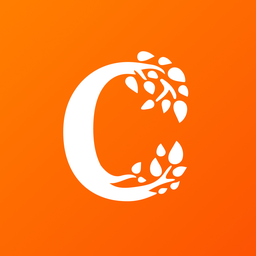
Full access? Get Clinical Tree
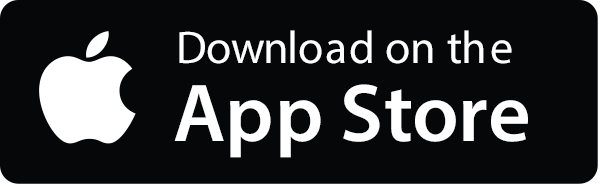
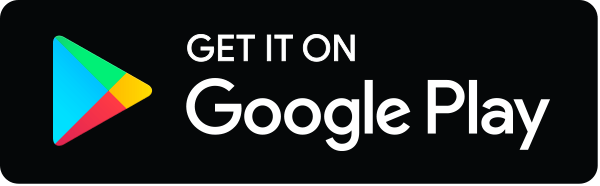