Introduction
The human immune system consists of many different cell types and organs that have evolved to destroy or control potentially harmful foreign substances. The immune response is essential for survival because it constitutes the principal means of defense against infection by pathogenic microorganisms, including those that enter and reside in the respiratory tract. The immune response is also critically involved in pathologic processes of the lung and upper respiratory tract. This chapter provides an understanding of the adaptive (or acquired) immune response, which depends on the specific recognition of antigens by T and Β lymphocytes. Immune recognition is highly specific for a particular pathogen, and yet an individual’s immune cells can collectively respond to an almost unlimited number of foreign antigens. The molecular mechanisms underlying this specificity and diversity are unique to the immune system. The adaptive immune response also changes after successive encounters with the same pathogen. For example, memory of an antigen allows the immune system to respond faster and in greater magnitude compared with the initial encounter. This chapter also describes how primary and secondary immune responses are regulated by complex cellular interactions and the release of particular types of soluble mediators. Antigen-specific immune responses are also regulated and augmented by nonspecific inflammatory cells of the immune system, such as dendritic cells, macrophages, neutrophils, eosinophils, and mast cells. Defects in the development of adaptive immunity are discussed in Chapter 92 on primary immunodeficiencies.
Components of the Immune System: Overview
All of the cells of the immune system arise from pluripotent hematopoietic stem cells through two main lines of differentiation that give rise to the lymphoid lineage and the myeloid lineage. Specificity within the immune system is primarily provided by lymphocytes. The two major categories of lymphocytes are T cells, which are derived from bone marrow stem cells and primarily develop in the thymus, and B cells, which develop in the bone marrow in adult humans. A third population of lymphocytes is natural killer (NK) cells.
Lymphocytes and other cells of the immune system express a large number of different molecules on their surfaces. Some of these markers can be used to separate cells with different functions or to distinguish cells at particular stages of differentiation. Monoclonal antibodies to many different cell surface markers have been produced, and a systematic nomenclature has been developed. The CD (“cluster of differentiation” or “cluster determinant”) system provides a basis by which monoclonal antibodies that bind to the same surface molecule are grouped together, and the CD number is used to indicate the specific molecule recognized. Tables 13-1 to 13-3 provide a partial list of surface antigens, particularly those mentioned in this chapter. The markers are partially grouped based on the cell type expressing them. It may be necessary to refer to this list of molecules throughout this chapter.
Cell Surface Markers | Identity/Function |
---|---|
TCR | Interacts with peptide/MHC complex on antigen-presenting cells |
CD2 | Binds to LFA3; involved in co-stimulation and adhesion |
CD3 | T-cell signaling complex |
CD4 | T-cell subset with helper function; interacts with MHC class II molecule |
CD8 | T-cell subset with cytotoxic function; interacts with MHC class I molecule |
CD25 | α chain of IL-2 receptor; expressed on activated T cells and on a subset of regulatory CD4 + T cells |
CD28 | Binds B7-1 (CD80) and B7-2 (CD86); co-stimulatory molecule involved in T-cell activation |
CD45 | Phosphatase involved in cellular activation and differentiation; different isoforms (CD45RA, CD45RO) mark naive versus previously activated T cells and stages of activation |
CD62L | L-selectin; involved in lymphocyte adhesion; levels mark naive versus memory cells |
CD69 | Activation marker |
CD95 (FAS) | FAS; receptor involved in apoptosis |
CD95L (FAS ligand) | Ligand for FAS; involved in T-cell–mediated killing |
CD152 (CTLA4) | Binds to B7-1 (CD80) and B7-2 (CD86); involved in down-regulation of TCR signaling |
CD154 (CD40 ligand) | Ligand for CD40; important for T-cell activation and T-cell–dependent B-cell activation |
CD134 (OX40), CD137 (4-1BB), ICOS, PD1 | Additional co-stimulatory molecules in the TNF or CD28 family; involved in T-cell activation and regulation |
Cell Surface Markers | Identity/Function |
---|---|
BCR | Immunoglobulin molecules; recognizes antigen |
CD5 | Binds to CD72; regulation of cell proliferation/activation; identifies B1a cell subset |
CD19 | B-cell coreceptor subunit; involved in co-stimulation |
CD20 | B-cell marker |
CD21 | Complement receptor type II; B-cell coreceptor subunit; marks certain B-cell subsets; EBV receptor |
CD22 | Adhesion molecule; involved in B-cell activation |
CD23 | Identifies B-cell subset; low-affinity receptor for IgE |
CD40 | Binds CD40L; involved in T-cell–dependent B-cell activation |
CD79a (Ig-α) | Involved in B-cell activation; signaling through BCR |
CD79b (Ig-β) | Involved in B-cell activation; signaling through BCR |
CD80 (B7-1) | Binds CD28 and CD152 (CTLA4) on T cells |
CD86 (B7-2) | Binds CD28 and CD152 (CTLA4) on T cells |
Cell Surface Markers | Distribution | Identity/Function |
---|---|---|
CD1 | Thymocytes, subset of lymphocytes, antigen-presenting cells | MHC class I–like molecule; involved in presentation of nonpeptide antigens |
CD11a | Leukocytes | α chain of LFA1; associates with CD18; interacts with ICAM1; involved in adhesion and migration |
CD11b | NK cells, monocytes, granulocytes | α chain of CR3; adhesion molecule |
CD11c | Monocytes, granulocytes | α chain of CR4; adhesion molecule; identifies dendritic cells |
CD14 | Granulocytes, monocytes | Receptor for LPS/LPB complex; myeloid differentiation antigen; cell activation |
CD16 | NK cells, monocytes | FCGR3; low-affinity receptor for IgG; involved in ADCC |
CD18 | Leukocytes | β chain of β 2 integrin molecules, including LFA-1, CR3, and CR4 |
CD29 | Leukocytes | β chain of β 1 integrin molecules, including VLA1-VLA6 |
CD32 | B cells, monocytes, granulocytes | FCGR3 |
CD35 | B cells, subset of NK cells, monocytes, granulocytes | CR1 |
CD45 | Leukocytes | Leukocyte common antigen; phosphatase; involved in cell signaling |
CD46 | Broad distribution | Membrane cofactor protein; regulates complement activation |
CD54 (ICAM1) | Broad distribution | Binds LFA1; adhesion molecule |
CD56 | NK cells | Neural cell adhesion molecule |
CD58 (LFA3) | Broad distribution | Binds CD2; adhesion molecule; involved in cell signaling |
T cells are distinguished by the presence of the T-cell receptor (TCR). Most T cells express a receptor composed of an α and a β chain, whereas a much smaller subset expresses a structurally similar receptor composed of a γ and a δ chain. Both receptors are associated with a complex of polypeptides, the CD3 complex, which provides a transmembrane signaling function and allows TCR engagement to be coupled to cellular activation. T cells expressing αβ TCRs can be divided into CD4 + and CD8 + T-cell subsets. CD4 + T cells primarily recognize antigens presented by major histocompatibility complex (MHC) class II molecules. CD8 + T cells primarily recognize antigens presented by MHC class I molecules. Functionally, T cells can be divided into several major subsets. For example, T helper cells may interact with B cells and help them to survive and divide, make antibody, and become memory B cells. T helper cells also may interact with cytotoxic T cells or with phagocytic cells and help them destroy intracellular pathogens. Different subsets of T helper cells can be distinguished by the pattern of cytokines that they secrete during an immune response. T helper cells are generally encompassed within the CD4 + T cell subset. Another subset of T cells is responsible for destruction of cells that have become infected by virus or other intracellular pathogens. These cells are called “cytotoxic T cells” and usually express the CD8 phenotypic marker. Although not clearly distinguished by phenotypic markers, separate subsets of T cells, within both the CD4 and CD8 subsets, have been termed “T regulatory (or suppressor) cells” because they down-regulate immune responses.
B cells are identified by the expression of surface immunoglobulin (Ig) or antibody molecules, which represent their specific B-cell receptor (BCR) for antigen. Analogous to the CD3 complex on T cells, BCRs are also linked to accessory molecules, Ig-α (CD79a) and Ig-β (CD79b), which are required for cellular activation after antigen interaction. After differentiation, B cells can develop the ability to produce high levels of antibody (soluble Ig). B cells also express a large number of other surface markers that are critically involved in their function and interaction with T cells. For example, most B cells express MHC class II molecules that allow them to present antigen to T helper cells. Other B-cell surface molecules are listed in Table 13-2 .
A third population of lymphocytes is probably best defined as those cells that do not express either TCR or Ig and mostly includes NK cells. A large proportion of this subset contains numerous electron-dense granules and is recognized morphologically as large granular lymphocytes. Markers on these cells are frequently shared with T cells (e.g., CD2 and CD8) or cells of the myelomonocytic series, for example, the integrin molecule CD11b or the low-affinity receptor for IgG (FCGR3 or CD16). NK cells appear to play an important role in the initial (innate) host defense against infection and tumor cells. Similar to certain phagocytes, they also have the capability to destroy target cells or pathogens that have been coated with specific antibody via a process known as antibody-dependent cellular cytotoxicity.
The myeloid lineage consists primarily of monocytes (macrophages) and neutrophils, which provide nonspecific inflammatory mediators and phagocytic function. These cells are critically involved in the nonspecific component of the inflammatory response (see Chapters 12 and 15 ). In addition, macrophages and certain other nonlymphoid cells, such as dendritic cells, are specialized to present antigens to T cells, thus contributing to specific immune responses.
The cells involved in the immune response are organized into tissues and organs. Primary lymphoid organs are the major sites of lymphopoiesis, in which stem cells and their committed precursor cells differentiate into lymphocytes and acquire specific functions. In humans, T lymphocytes mainly develop in the thymus, and B lymphocytes develop in the fetal liver and adult bone marrow. In the thymus, T-cell differentiation also includes acquiring the ability to recognize foreign antigens in the context of self-MHC molecules and the elimination of self-reactive cells (self-tolerance). B-cell acquisition of self-tolerance during development appears to take place in the bone marrow.
Differentiated lymphocytes migrate to secondary lymphoid organs, which include lymph nodes, spleen, and mucosa-associated lymphoid tissues, such as the tonsils, lymph nodes of the respiratory tract, and Peyer patches of the gut. These tissues provide an environment for lymphocytes to interact with each other, with antigen-presenting cells and other accessory cells, and with foreign antigens. The immune response is generated mostly within these secondary lymphoid organs, and lymphocytes migrate through the blood and lymph from one lymphoid organ to another and to nonlymphoid tissues. For example, foreign antigen exposure in the lung usually involves the movement of antigen to surrounding lymph nodes, where the specific immune response by T and B cells takes place. Generation of a cell-mediated immune response or antibody response allows antigen-specific effector T cells or specific antibodies, respectively, to travel back to lung tissue for a direct assault on foreign antigens.
Under normal conditions, there is a continuous active flow of lymphocyte traffic through the lymph nodes. About 1% to 2% of the lymphocyte pool recirculates each hour, allowing a large number of antigen-specific lymphocytes to come into contact with their appropriate antigen. Recirculating lymphocytes leave the blood and enter the lymph node through specialized postcapillary venules, known as high endothelial venules (HEVs). Specific interacting receptors on lymphocytes and HEV cells facilitate this homing process. Lymphocytes return to the circulation by way of afferent lymphatics that pass via the thoracic duct into the left subclavian vein. Lymphocytes also enter mucosa-associated lymphoid tissues, such as the tonsils and Peyer patches, via HEVs. The recirculation and trafficking of memory and effector T and B cells is tightly regulated, determined by unique combinations of adhesion molecules and chemokines. For example, certain lymphocytes may preferentially migrate across HEVs into intestinal lymphoid tissues (either Peyer patches or mesenteric lymph nodes) or into respiratory tract tissues or may specifically home to the peripheral lymph nodes or the spleen.
Separate combinations of cell-surface adhesion molecules and chemokines allow activated lymphocytes and other leukocytes to migrate into nonlymphoid tissues, especially during inflammation and in response to the release of inflammatory cytokines. The difference in trafficking patterns for nonactivated (resting) versus activated lymphocytes is striking and emphasizes the importance of particular adhesion molecules in the control of lymphocyte migration.
Immune Recognition
B Cells and Antibodies
Structure of Immunoglobulin and the B-Cell Receptor for Antigen
Immunoglobulin molecules, or antibodies, are glycoproteins that act as BCRs. These molecules can also be secreted in large quantities by activated B cells and plasma cells. Figure 13-1 shows the basic structure of an Ig molecule. Each Ig molecule is bifunctional—one region (Fab) binds to antigen, and a different region (Fc) mediates various effector functions, such as binding to host tissues (via their cell surface Fc receptors) and binding to and activating the first component of the classic complement system.

The basic structure of all Ig molecules involves two identical light polypeptide chains and two identical heavy polypeptide chains linked together by disulfide bonds (see Fig. 13-1A ). The isotype (class or subclass) of an Ig molecule is determined by its heavy chain type. There are five Ig classes—IgG, IgM, IgA, IgD, and IgE—corresponding to the γ, µ, α, δ, and ε heavy chain types. In humans, there are four IgG subclasses, IgG1 to IgG4. There are major differences in the structure and main functions of these different Ig classes and subtypes.
In Figure 13-1B , the IgG molecule is shown as an example of basic antibody structure. Each chain is composed of a series of globular regions or domains. Each domain encompasses about 60 to 70 amino acids and has an internal disulfide bond. The site of antigen binding is the amino (NH 2 )-terminal domain for both the heavy (H) and light (L) chains. This domain is characterized by remarkable sequence variability and is referred to as the “variable region” of the heavy and light chains (V H and V L region, respectively). The combination of V H and V L forms the antigen-binding site, and there are two such sites per IgG molecule (see Fig. 13-1B ). The rest of each polypeptide has a relatively constant structure. The constant domain of the light chain is termed the “C L region,” whereas the heavy chain has three constant domains: C H 1, C H 2, and C H 3. The hinge region, located between the C H 1 and C H 2 domains, provides flexibility and independence to the two antigen-binding sites. In both µ and ε heavy chains, there is an additional constant domain between C H 1 and C H 2, resulting in a total of four constant domains.
The greatest variability in antibody molecules takes place in the V H and V L domains, and these domains are responsible for the specificity in antigen binding. Within the variable domains (see Fig. 13-1C ), certain short segments show exceptional variability and are called “hypervariable regions.” These regions are also referred to as complementarity-determining regions (CDRs) because they are directly involved in the binding to antigen. In both the V H and V L regions, there are three CDRs (CDR1 to CDR3) with intervening segments referred to as “framework regions” (see Fig. 13-1C ). CDR3 (a component of the polypeptide V region) is formed by parts of the V (variable), D (diversity), and J (joining) gene segments. Variation within the V H and V L regions distinguishes one antibody molecule from another and is referred to as “idiotype” or “idiotypic variation . ” Additional information on heavy chain rearrangement is shown in eFig. 13-1 .
Formation of the B-Cell Receptor Repertoire
The BCR repertoire and the Ig molecules are characterized by enormous diversity. The principal genetic mechanisms that are used to generate this diversity include (1) genetic recombination of gene segments to form a functional Ig gene, (2) combinatorial diversity of heavy and light chain matching, and (3) somatic mutation of rearranged genes. Another major force in shaping the antibody repertoire has been termed “receptor editing , ” which allows receptors with self-reactive potential to be modified by additional recombination events during B-cell differentiation.
Figure 13-2A illustrates the principle of genetic recombination for an Ig gene. In this case, one of several variable (V) region gene segments (normally separated upstream from the constant [C] region gene segments on the same chromosome) can be linked to a single C region gene segment by genetic recombination. The combining of gene segments, rather than the existence of a single gene coding for every individual antibody molecule, considerably reduces the amount of genetic information required to encode many different antibody molecules. In Figure 13-2B the different gene segments that encode a κ light polypeptide chain are shown. Note that in this case, a V region rearranges proximal to a J segment, which is linked to the C region segment. Rearrangement of κ or γ light chain genes involves V, J, and C region gene segments (see Fig. 13-2A ; see also Fig. 13-1C ). In the formation of a functional heavy chain gene, a successful rearrangement of gene segments includes one V, one D, and one J region segment linked to a C region segment (compare Figs. 13-1C and 13-2B ).

During genetic recombination, additional variability is generated by a process known as “junctional diversification.” When two gene segments are brought together during rearrangement, the linking is not precise. Instead, some nucleotides are inserted or deleted randomly at the junctional site. This introduces new codons, and therefore new amino acids, into the junctional sequence. If an incorrect number of junctional nucleotides are added or deleted, functional molecules will not be encoded, because the rest of the gene will be “out of frame” or a “stop” sequence will be introduced. Junctional diversification takes place in the hypervariable CDR3 region of the molecule (see Fig. 13-1C ).
In general, a single B cell usually expresses an Ig molecule of only one antigen specificity, composed of one heavy chain molecule and one light chain molecule. Even though there are two chromosomal copies of the heavy chain genes in each cell, only one is usually functionally expressed. This phenomenon of using genes on only one parental chromosome is known as “allelic exclusion.” Once there has been a functional rearrangement of heavy chain genes on one parental chromosome, rearrangement of heavy chain genes on the other chromosome is mostly prevented. If the first rearrangement is not successful, the second one can take place. All of the genetic events described earlier happen before the B cell encounters its antigen. The B-cell (or antibody) response diversifies further after interaction with antigen by a process known as “somatic mutation,” primarily involving point mutations in the hypervariable regions of the V regions. Somatic mutation can be viewed as “fine tuning” of the antibody response, occurring after the primary response to a stimulus and during the development of memory B cells. It is, therefore, mostly observed during a secondary immune response. Somatic mutation allows for the production of antibodies with higher affinity for antigen, that is, the ability to bind more strongly to an antigen. Although the mutations are random, B cells with high affinity are selectively expanded (referred to as “affinity maturation”) because the stronger binding allows preferential stimulation by the target antigen. Somatic mutation is closely tied to isotype switching, and T-cell help is required. Somatic mutation usually takes place at the site of T-cell–B-cell interaction in the germinal centers of lymph node or spleen.
Isotype Switching and Function of the Different Immunoglobulin Classes
One B cell usually makes antibody of a single specificity that is fixed by the nature of V L J L and V H D H J H rearrangements. During the lifetime of this cell, however, it can switch from making an IgM molecule to producing a different class of antibody, such as IgG or IgA, while retaining the same antigenic specificity. This phenomenon is known as “class switching” or “isotype switching.”
Figure 13-3 shows the predominant mechanism by which a B cell switches from production of surface IgM to secretion of an IgG, IgA, or IgE molecule. The mechanism involves further DNA rearrangement (a process unique to Ig heavy chain genes), linking rearranged VDJ gene segments with a different heavy chain C region gene segment downstream. The switch (S) region is a stretch of repeating sequences 5′ to the C region that allows a VDJ unit (previously linked to the Cµ region gene segment) to rearrange to another C region downstream. In the process the intervening DNA is deleted. The B cell therefore cannot return to IgM production. Class switching generally follows stimulation of the B cell by antigen and frequently is dependent on factors released by T helper cells.

Each class of secreted Ig has a different set of functions ( Table 13-4 ). IgG is the major antibody of secondary immune responses and is most important for obtaining effective immunization to various toxins, toxoids, and certain extracellular pathogens. IgG accounts for 70% to 75% of the total Ig pool and is the major Ig class in normal human serum. It also crosses the placenta and confers immunity to newborns and neonates for the first few months of life. The interaction of IgG antibodies with antigen can have numerous consequences, including precipitation, agglutination, complement activation, and various cellular effector functions via IgG receptors. There are four IgG subclasses, with IgG1 and IgG3 being most effective at fixing complement and activating complement-mediated effector functions.
Effector Function | IgM | IgD | IgGl | IgG2 | IgG3 | IgG4 | IgA | IgE |
---|---|---|---|---|---|---|---|---|
Mean serum levels (mg/mL) | 1.5 | 0.04 | 9 | 3 | 1 | 0.5 | 2.1 | 0.00003 |
Opsonization | — | — | +++ | — | ++ | + | + | — |
Complement fixation | +++ | — | ++ | + | +++ | — | — | — |
Binding of Fc receptor on phagocytic cells | — | — | ++ | ++ | + | — | — | |
Induction of mast cell degranulation | — | — | — | — | — | — | — | +++ |
* Activity levels of immunoglobulin classes and subclasses: +++ high; ++ moderate; + low; =/— minimal; — none.
IgM is the predominant early-secreted antibody, frequently seen in primary immune responses. IgM is important for effective responses to antigenically complex infectious organisms, especially those with polysaccharide-containing cell walls. IgM antibodies may also be extremely effective at precipitation, agglutination, and complement activation after binding to antigen. Secreted IgM is usually found as a pentamer of the basic (four-domain) Ig unit. Polymerization is facilitated by the binding of the heavy chain tailpieces to a joining (J) peptide.
IgA plays a major role in mucosal immunity and is the predominant Ig in secretions such as saliva and tracheobronchial secretions. Secretory IgA exists mainly in dimeric form and contains a secretory component, which is synthesized by epithelial cells and facilitates transport into secretions as well as protection from proteolysis. Secretory IgA is involved in the prevention of microbial adherence to mucosal cells and in the agglutination of microorganisms. IgA provides the first line of defense against a variety of pathogens.
IgE is scarce in the serum. Its major importance relates to its ability to bind to FcεR1 receptors on mast cells and basophils, and cross-linking of IgE bound to these cells results in cellular activation, degranulation, and release of mediators involved in allergic responses, such as histamine and various leukotrienes. IgE plays a role in immunity to parasites, but in developed countries, it is more commonly associated with allergic responses and allergic diseases, such as hay fever and asthma (see Chapters 41 and 42 ).
B-Cell Development
B cells are produced in the specialized microenvironments of the fetal liver and the adult bone marrow. After migration out of the bone marrow, the life span of mature naive B cells is limited unless there is contact with antigen. B cells interact with foreign antigen in the peripheral lymphoid tissues, particularly in the germinal centers of lymph nodes and spleen. The interaction with antigen, in the setting of T-cell help, results in the generation of memory B cells and cells that secrete a large amount of Ig. This frequently involves class switching and somatic mutation, which allows a secondary antibody response to generate antibodies of a different isotype with higher affinity for the stimulating antigen. An additional view of B-cell development is shown in eFig. 13-2 .
The formation of the B-cell repertoire in the bone marrow is mostly random, and B cells with self-reactivity are generated. Negative selection of cells capable of strongly binding to self-antigens is an important part of B-cell development. This process of B-cell self-tolerance involves both deletion (elimination) and functional inactivation (anergy) of self-reactive cells. In addition, B cells with specificity for self-antigens can modify their receptors through a process called “receptor editing . ” These self-reactive B cells undergo a reversible arrest of development and reinitiate light chain gene rearrangements to alter their BCR. If a B cell fails to edit its BCR, it is destined for cell death (apoptosis). Immature B cells in the bone marrow appear to be capable of receptor editing, whereas mature B cells in the peripheral lymphoid tissues normally lose this ability to initiate a new round of Ig gene rearrangements.
Immunoglobulin Interactions with Antigen
An antigen is a molecule or molecular complex recognized by B cells or T cells. The term immunogen usually refers to a substance capable of eliciting an immune response, and therefore it must also be capable of being recognized as an antigen. An antigen (e.g., a protein molecule) is usually much larger than the small region fitting into the binding site of an Ig molecule or the processed peptide recognized by a TCR. This smaller region is frequently referred to as an “antigenic determinant” or “epitope . ” In a protein a B-cell epitope can theoretically be constructed in two ways—as a continuous or a discontinuous epitope. In a continuous epitope, the amino acid residues are part of a single uninterrupted sequence, whereas in a discontinuous epitope, residues are not contiguous in the primary structure but are brought together by the folding of the polypeptide chain. Because this kind of epitope requires a special conformation of the antigen, it is frequently referred to as a “conformational epitope.”
B cells and T cells usually recognize different parts of an antigen. B cells and their secreted Ig molecules most commonly recognize unprocessed or “native” antigens. These antigens have maintained their native configuration, and most of the epitopes are usually of the discontinuous or conformational type. In general, only a minor component of a B-cell response is directed to small linear peptide regions of the antigen. Studies indicate that epitopes recognized by B cells are not randomly distributed throughout the antigen but rather reside in regions with particular structural features. One important feature is “accessibility,” because epitopes normally must be on the outer surface of a protein and may protrude from an antigen’s globular surface to be able to interact with the BCR.
T Cells and Antigen-Presenting Cells
In contrast to B cells, T cells recognize processed pieces of a protein antigen, which are presented to the TCR by MHC molecules on the surface of antigen-presenting cells.
T-Cell Receptors
The TCR shows important structural similarities with Ig molecules ( Fig. 13-4 ). The αβ TCR is expressed by at least 90% of peripheral blood T cells. Essentially, all CD4 + T cells and most CD8 + T cells express this form of the TCR. A small percentage of αβ-expressing T cells have a double-negative (CD4 − and CD8 − ) phenotype. As shown in Figure 13-4 , each chain consists of two extracellular Ig-like domains anchored into the plasma membrane by a transmembrane region and a short cytoplasmic tail. Similar to Ig molecules, the outer NH 2 – terminal domain of each chain constitutes the variable region. Outside of the transmembrane region, the two chains are covalently linked together by disulfide bonds. Due to the short cytoplasmic tail, the αβ heterodimer is not capable of transmitting an intracellular signal after TCR engagement. This function is accomplished by the CD3 complex of polypeptides and other signaling proteins that are associated with the TCR.

The γδ TCR is an alternative form of TCR that is similar in overall structure to the αβ receptor. Although some γδ cells express CD8, most are CD4 − and CD8 − (double negative). CD8 expression is largely confined to those γδ cells residing in the small intestine. The γδ TCR is also expressed in association with the CD3 complex. Although γδ T cells form a minor proportion of the T cells in the thymus and secondary lymphoid organs, they are abundant in various intraepithelial locations, such as in the skin, intestines, and lung.
T-Cell Receptor Structure and T-Cell Receptor Repertoire Formation
Genes encoding the TCR are organized similarly to Ig genes ( eFig. 13-3 ). In a manner similar to that described previously for B cells, rearrangement of gene segments, junctional diversity, and combinatorial joining of the two chains is responsible for the diversity of the TCR repertoire. However, in contrast to B cells, TCR genes do not undergo somatic mutation. Thus nearly the entire TCR αβ repertoire is formed during T-cell development in the thymus and before any interaction with antigen. Because the T-cell repertoire is shaped to recognize self-MHC antigens, it is believed that extrathymic somatic mutation might result in the generation of deleterious self-reactive T cells.
The components of the αβ TCR heterodimer are shown in Figure 13-4 , and the ribbon backbone structure of the Vα and Vβ portions of a human TCR are shown in Figure 13-5 . The upward-pointing loop structures are the CDRs. These regions are the most variable part of the TCR α and β chains and are most important for binding of the TCR to the MHC/peptide complex. The CDR1 and CDR2 regions of the α chain and β chain are encoded within the germline TCRAV and TCRBV gene segments, and variability in their sequences distinguishes the different V region subfamilies. The most diverse part of the α and β chains is the CDR3, which directly interacts with peptide in the binding groove of the MHC molecule.

Antigen-Presenting Cells and the Major Histocompatibility Complex
There are two major varieties of MHC molecules (and genes) involved in the presentation of antigens to T cells. MHC class I molecules include human leukocyte antigen (HLA)-A, HLA-B, and HLA-C molecules. MHC class II molecules include the HLA-DR, HLA-DQ, and HLA-DP molecules. Class I molecules are expressed on nearly all nucleated cells. In contrast, class II molecules have a limited distribution and are normally present only on cells involved in antigen presentation to T cells, including dendritic cells, macrophages, B cells, and thymic epithelial cells. The limited expression of class II antigens in different tissues may be extremely important in preventing various types of autoimmune reactions. After activation or exposure to certain cytokines, such as interferon-γ (IFN-γ), other human cell types such as activated T cells and epithelial cells can express class II molecules.
The general structures of the two classes of MHC molecules are shown in Figure 13-6 . For MHC class I antigens, the α chain (encoded within the MHC) is complexed to beta 2 -microglobulin (encoded outside the MHC). The α chain is highly polymorphic (variable between individuals), whereas beta 2 – microglobulin is invariant. The extracellular portion of the α chain is divided into three domains: α1, α2, and α3. The outer α1 and α2 domains represent the polymorphic components of the molecule, and the α3 domain is relatively constant. MHC class II molecules are composed of an α and a β chain, both of which are encoded within the MHC. The NH 2 -terminal domains of each chain (α1 and β1 domains) represent the polymorphic regions of the molecule and are important in antigen presentation, whereas the α2 and β2 domains are relatively constant.

The structures of MHC class I and class II molecules have provided remarkable insight into how antigenic peptides are bound and presented to T cells. In Figure 13-7A , the peptide-binding groove of a class I molecule is viewed from the top, showing the surface that is contacted by a TCR. MHC class I pockets can usually only bind peptides of 8 to 10 amino acids, which are bound in a typical extended conformation with both the NH 2 terminus and the carboxy (COOH) terminus anchored in the peptide-binding groove. In the case of MHC class II, the peptide-binding groove is formed by the interaction of the NH 2 -terminal domains of the α and β chains (see Fig. 13-7B ). The structure of MHC class II allows peptides of varying lengths to bind because both ends of the peptide are free, and the peptide shown in Fig. 13-7B has the typical polyproline-like extended helical conformation seen in all class II MHC-bound peptides.
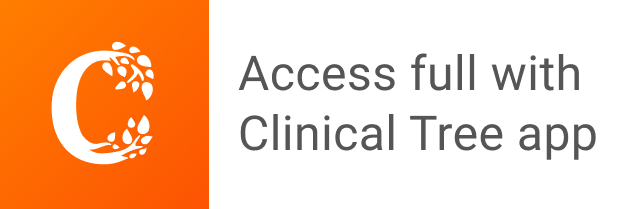