Introduction
This first chapter in the section on respiratory physiology is devoted to the primary function of the lung: gas exchange. In addition, the principles of ventilation and blood flow that underlie gas exchange are reviewed. Although the lung has other functions, such as metabolizing some compounds, filtering unwanted materials from the circulation, and acting as a reservoir for blood, gas exchange is its chief function. Respiratory diseases frequently interfere with ventilation, blood flow, and gas exchange and may ultimately lead to respiratory failure and death.
Ventilation
The anatomy of the airways and the alveolar region of the lung is discussed in Chapter 1 . There we saw that the airways consist of a series of branching tubes that become narrower, shorter, and more numerous as they penetrate deeper into the lung. This process continues down to the terminal bronchioles, which are the smallest airways without alveoli. All these bronchi make up the conducting airways. Their function is to channel inspired gas to the gas-exchanging regions of the lung. Because the conducting airways contain no alveoli and therefore take no part in gas exchange, they constitute the anatomic dead space.
Each terminal bronchiole subtends a respiratory unit, or acinus. The terminal bronchioles divide into respiratory bronchioles that have occasional alveoli budding from their wall, and these then transition to the alveolar ducts, structures that are completely lined with alveoli. This alveolated region of the lung where gas exchange takes place is known as the respiratory zone. The distance from the terminal bronchiole to the most distal alveolus is only approximately 5 mm, but the respiratory zone makes up most of the lung in terms of gas volume (some 2 to 3 L).
The morphologic characteristics of the human airways were greatly clarified by Weibel. He measured the number, length, width, and branching angles of the airways, and he proposed models that, although they are idealized, make pressure-flow and other analyses much more tractable.
The most commonly used Weibel model is the so-called model A, shown in Figure 4-1 . Note that the first 16 generations (Z) make up the conducting airways ending in the terminal bronchioles. The next three generations constitute the respiratory bronchioles, in which the degree of alveolation steadily increases. This is called the transitional zone because the nonalveolated regions of the respiratory bronchioles do not have a respiratory function. Finally, there are three generations of alveolar ducts and one generation of alveolar sacs. These last four generations constitute the true respiratory zone .

Other models of the airways have been proposed. However, the Weibel model has been of great value to respiratory physiology, and an example of its use is shown in Figure 4-2 . Here the model clarifies the nature of gas flow in all generations of the airways in the lung. Figure 4-2 shows that, if the total cross-sectional area of the airways of each generation is calculated, there is relatively little change in area until we approach generation 16, that is, the terminal bronchioles. However, near this level, the cross-sectional area increases very rapidly. This has led some physiologists to suggest that the shape of the combined airways is similar to a trumpet or even a thumbtack!

The result of this rapid change in area is that the mode of gas flow changes in the region of the terminal bronchioles. Proximal to this point, flow is convective, or “bulk,” that is, similar to the sort of flow that results when beer is poured out of a pitcher. However, when the gas reaches the region approximating the level of the terminal bronchioles, its forward velocity decreases dramatically because of the very sudden increase in cross-sectional area. As a consequence, diffusion begins to take over as the dominant mode of gas transport. Naturally, there is no sharp transition; flow changes gradually from primarily convective to primarily diffusive in the general vicinity of generation 16.
One implication of this change in mode of flow is that many aerosol particles penetrate to the region of the terminal bronchioles by convective flow, but they do not penetrate further because of their large mass and resulting low diffusion rate. Thus sedimentation of these particles is heavy in the region of the terminal respiratory bronchioles. This is one reason why this region of the lung is particularly vulnerable to the effects of particulate air pollutants.
Another implication of this dichotomously branching airway tree is that the greater the number of branch points, the greater the potential for nonuniform distribution of airflow among the distal airways and alveoli. In addition, repeated, possibly minor, differences in flow distribution at each branch point will give rise to spatial correlation of flow; in other words, neighboring regions will tend to have more similar flows than regions located far apart, other factors being equal.
Lung Volumes
Figure 4-3 shows the major divisions of lung volume. Total lung capacity is the volume of gas contained in the lungs at maximal inspiration. The vital capacity is the volume of gas that can be exhaled by a maximal expiration from total lung capacity. The volume remaining in the lung after maximal expiration is the residual volume (RV). Tidal volume refers to the normal respiratory volume excursion. The lung volume at the end of a normal expiration is the functional residual capacity (FRC). The diagram also indicates the inspiratory reserve volume and the expiratory reserve volume . These volumes change in characteristic directions with different respiratory diseases, and so their measurement becomes important. Indeed, they have for years formed the basis for diagnosis and monitoring of chronic diseases such as chronic obstructive pulmonary disease (COPD) and lung fibrosis (see Chapter 25 ).

Functional Residual Capacity, Residual Volume, and Total Lung Capacity
These three volumes cannot be measured with a spirometer (a device that measures the volume of air being exhaled or inhaled) because there is no way of knowing the volume remaining in the lung after a maximal expiration (i.e., the RV). However, if any one of these three volumes is measured by an independent method, the other two can be derived by spirometry.
The FRC can be measured conveniently by helium dilution in a closed circuit. The subject is connected to a spirometer of known volume that contains a known concentration of helium (a very insoluble gas) and then rebreathes until the helium concentration in the spirometer and in the lungs is the same. The exhaled carbon dioxide is absorbed with soda lime, and oxygen is added to maintain a constant total volume. After equilibration, the total amount of helium is assumed to be unchanged because so little of it is removed by the blood because of its very low solubility. The FRC can then be derived from the following equation that expresses this conservation of mass principle:
C 1 × V 1 = C 2 × ( V 1 + V 2 )
Another common way of measuring the FRC is with a body plethysmograph. This is a large airtight box in which the subject sits. At the end of a normal expiration, a shutter closes the mouthpiece, and the subject is asked to make respiratory efforts. As the subject tries to inhale, the gas in the lungs expands, lung volume increases slightly, and the pressure in the box rises slightly because its gas volume decreases. Boyle’s law (pressure times volume is constant at constant temperature) can then be used to calculate the change of volume of the plethysmograph. The equation is P 1 V 1 = P 2 (V 1 − ΔV), where P 1 and P 2 are the box pressures before and after the inspiratory effort, V 1 is the preinspiratory box volume, and ΔV is the change in the volume of the box (or lung). If mouth pressure is also measured during the respiratory efforts, Boyle’s law can also be applied to the lung and FRC can be derived. The equation here is P 3 V 2 = P 4 (V 2 + ΔV), where P 3 and P 4 are the mouth pressures before and after the inspiratory effort, and V 2 is the FRC. Because this is the only unknown (ΔV was measured previously), V 2 can be calculated.
In patients with lung disease the FRC measured by helium dilution may be substantially less than that measured by body plethysmography. The reason is that the body plethysmograph measures the total volume of gas in the lung, including any that is trapped behind closed airways (i.e., unventilated regions that contain gas). By contrast, the helium dilution method measures only ventilated lung regions. In young normal subjects, these volumes are virtually identical, but they may be considerably different in patients with severe lung disease. Also in these patients, regions that are poorly ventilated reduce the overall speed of equilibration of helium, which will lead to volume underestimation if rebreathing is stopped too soon.
Total and Alveolar Ventilation
Total Ventilation
Total ventilation, also called minute ventilation, is the total volume of gas exhaled per minute. It is equal to the tidal volume times the respiratory frequency. The volume of inhaled air is slightly greater than the exhaled volume because more oxygen is inhaled than carbon dioxide is exhaled, but the difference is usually less than 1%.
Alveolar ventilation is the amount of fresh inspired air (non–dead space gas) that enters the alveoli per minute and is therefore available for gas exchange. Strictly, the alveolar ventilation is also measured during expiration, but the inhaled and exhaled volumes are almost the same.
Alveolar Ventilation
Because the tidal volume (V t ) is made up of the dead space volume (V d ) and the volume of gas entering (or coming from) the alveoli (V a ), the alveolar ventilation can be measured from the following equations:






A difficulty with this method is that the anatomic dead space is not easy to measure, although a value for it can be assumed with little error. One milliliter per pound of body weight is a common assumption. This approximation will overestimate dead space in obese subjects and so should be applied using ideal body weight for height.
Another way of measuring alveolar ventilation in normal subjects is to use the alveolar ventilation equation , which expresses mass conservation of carbon dioxide by defining carbon dioxide production ( ) as the product of alveolar ventilation (
) and fractional alveolar concentration of carbon dioxide (F aco 2 ). Because concentration is proportional to partial pressure, the relationship can be written as:







Anatomic Dead Space
The anatomic dead space is the gas volume contained within the conducting airways. The normal value is in the range of 130 to 180 mL and depends on the size and posture of the subject. The value increases slightly with large inspirations because the radial traction exerted on the bronchi by the surrounding lung parenchyma increases their size. Anatomic dead space can be measured by Fowler’s method, in which a single breath of oxygen is inhaled and the concentration of nitrogen in the subsequent expiration is analyzed, as shown in Figure 4-4 .

Physiologic Dead Space
Unlike anatomic dead space, which is determined by the anatomy of the airways, physiologic dead space is a functional measurement based on the ability of the lungs to eliminate carbon dioxide. It is defined by the Bohr equation:


Inequality of Ventilation
Not all the alveoli are equally ventilated, even in the normal lung. There are several reasons for this, related both to gravitational (topographic) and to nongravitational influences on gas distribution.
Topographic Inequality
Regional differences in ventilation can be measured by having the patient inspire a radioactive gas such as xenon ( 133m Xe). In one technique the patient inhales a single breath of gas, and its concentration is detected by a radiation camera placed behind the chest. An additional measurement is made after the patient has rebreathed long enough to allow the xenon to equilibrate throughout the different regions of the lungs, thus reflecting regional lung volumes. By comparing the first and the second measurements, the ventilation per unit alveolar volume can be obtained.
Measurements in upright normal subjects show that the ventilation per unit volume of the lung is greatest near the base of the lung and becomes progressively smaller toward the apex. When the subject lies supine, this difference becomes much less, but the ventilation of the lowermost (posterior) lung exceeds that of the uppermost (anterior). In the lateral decubitus position, again, the dependent lung is better ventilated. (These results refer to an inspiration from FRC.)
An explanation of this topographic inequality of ventilation is shown in Figure 4-5A , which depicts conditions at FRC. The intrapleural pressure is less negative at the bottom than at the top of the lung. This pattern can be attributed to the weight of the lung, which requires a larger pressure below the lung than above it to balance the downward-acting weight forces. There are two consequences of this lower expanding pressure on the base of the lung. First, the resting volume of the basal alveoli is smaller, as shown by the pressure-volume curve. Second, the change in volume for a given change in intrapleural pressure is greater because the alveoli are operating on a steeper part of the pressure-volume curve. Thus the ventilation (change in volume per unit resting volume) is greater at the base than the apex. However, if a normal subject makes a small inspiration from RV (rather than from FRC), an interesting change in the distribution of ventilation is seen. The major share of the ventilation goes to the apex of the upright lung, whereas the base is very poorly ventilated. Figure 4-5B shows why a different pattern is seen in this case. Now the intrapleural pressures are less negative, and the pressure at the base of the lung actually exceeds atmospheric pressure. For a small fall in intrapleural pressure, no gas will enter the extreme base of the lung, and only the apex will be ventilated. Thus the normal pattern of uneven ventilation is reversed in this early phase of inhalation.

Hyperpolarized helium and xenon magnetic resonance imaging have been used to measure regional ventilation and give similar results, although there are small differences in results obtained with the two gases for reasons that remain to be determined.
Airway Closure
At RV the compressed region of the lung at the base in Figure 4-5B does not have all its gas squeezed out because small airways, probably in the region of the respiratory bronchioles, close first and trap gas in the distal alveoli. This is known as airway closure. In young normal subjects, airways close only at lung volumes below FRC. However, in older normal subjects, the volume at which the basal airways close ( closing volume ) increases with age and may encroach on the FRC. The reason for this increase is that the aging lung loses some of its elastic recoil and the intrapleural pressures therefore become less negative, thus approaching the situation shown in Figure 4-5B . Under these conditions, basal regions of the lung may be ventilated only intermittently, with resulting defective gas exchange. A similar situation frequently develops in patients with COPD in whom lung elastic recoil may be reduced.
Nontopographic Inequality
In addition to the topographic inequality of ventilation caused by gravitational factors (see Fig. 4-5 ), nongravitational mechanisms also exist. This is proved by the fact that even astronauts in space beyond the reach of gravity show uneven ventilation by both the single-breath and the multibreath nitrogen washout methods. These methods are described in Chapter 25 . Such findings have been confirmed by studies in which inspired gas is labeled by small particles. Such studies show considerable variability in ventilation at a given horizontal level.
Several factors are responsible for uneven ventilation in the distal, smaller regions of the lung. One of these is the existence of uneven time constants. The time constant of a region of lung is given by the product of its resistance and compliance (analogous to the time constant in electrical circuits, which is the product of electrical resistance and capacitance). Lung units with different time constants inflate and deflate at different flow rates. Depending on the breathing frequency, a unit with a large time constant does not complete its filling before expiration begins and therefore is poorly ventilated; the faster the frequency, the less time for ventilation. In contrast, a unit with a small time constant, which fills rapidly, may receive a higher proportion of gas from the anatomic dead space, which also reduces its effective alveolar ventilation.
Another cause of uneven ventilation in small lung units is the asymmetry of their structure, which can result in a greater penetration of gas by diffusion into the smaller units than into the larger. The resulting somewhat complex behavior is known as diffusion- and convection-dependent inhomogeneity and may play an important role in lung disease.
A further possible reason for uneven ventilation at the level of small lung units is the presence of concentration gradients along the small airways. This is known as series inequality. Recall that inspired gas reaches approximately the region of the terminal or respiratory bronchioles by convective flow, but gas flow over the rest of the distance to the alveoli is accomplished principally by diffusion within the airways. If there is abnormal dilatation of an airway, the diffusion process may not be complete within the breathing cycle, and the distal alveoli will be less well ventilated than the proximal alveoli.
Blood Flow
Blood flow is as important for gas exchange as is ventilation. This has not always been appreciated, partly because the process of ventilation is more obvious, especially in the dyspneic patient, and is more accessible to measurement. Much has been learned about the pulmonary circulation in the past few years, especially its metabolic functions. The anatomy and function of the pulmonary circulation is also described in Chapters 1 and 6 .
Pressures of the Pulmonary Circulation
The pressures in the pulmonary circulation are very low compared with those in the systemic circulation, and this feature is responsible for much of its special behavior. The normal pressures in the human pulmonary artery are typically approximately 25 mm Hg systolic, 8 mm Hg diastolic, and 15 mm Hg mean. Normal mean systemic arterial pressure is approximately 100 mm Hg, which is six times higher than that in the pulmonary circulation. The evolutionary force to keep the pressures in the pulmonary circulation so low is the mechanical vulnerability of the extremely thin blood-gas barrier. Higher pressures in the pulmonary capillaries would cause stress failure of the capillary wall.
Pressure Inside Blood Vessels
Because the pulmonary arterial pressure is so low, hydrostatic effects within the pulmonary circulation are very important. The adult upright human lung is some 30 cm high, giving a hydrostatic difference in pressure of 30 cm blood between the extreme apex and the base, which is equivalent to approximately 23 mm Hg. As a result, there are very substantial differences in flow within the small pulmonary arteries and the capillaries between the top and bottom of the upright lung. This topic is discussed further in the section on the distribution of pulmonary blood flow.
Various techniques have been used to determine the pattern of pressure drop along the pulmonary blood vessels. These include measurement of the transudation pressure on the pleural surface of isolated lung, measurement of the pressure transient resulting from the injection of a slug of low- or high-viscosity blood into the pulmonary artery, and direct puncture of different-sized vessels along with direct measurement of hydrostatic pressure. The direct puncture measurements indicate that much of the normal pressure drop in the pulmonary circulation probably takes place in the pulmonary capillaries, and that the mean capillary pressure is approximately halfway between that in the pulmonary artery and that in the pulmonary vein ( Fig. 4-6 ). This distribution of the pressure drop is consistent with the main function of the pulmonary circulation, which is to expose as large an area of blood as possible to the alveolar gas.

The distribution of pressure along the pulmonary blood vessels depends on lung volume. At low states of lung inflation, the resistance of the extra-alveolar vessels (see next section) increases, and more pressure drop then takes place across the pulmonary arteries and veins. By contrast, there is evidence that, at very high states of lung inflation, the resistance of the capillary bed is increased, and therefore there will be an additional pressure drop in the capillaries.
The pressures in the pulmonary circulation are highly pulsatile; indeed, if we take the normal systolic and diastolic pressures in the main pulmonary artery as 25 and 8 mm Hg, respectively, this represents a much greater proportional change than the systolic-diastolic difference in systemic arteries (120 and 80 mm Hg, respectively). There is good evidence that the pulsatility of pressure, and therefore flow, extends to the pulmonary capillaries.
Pressures Outside Blood Vessels
Some pulmonary blood vessels are exposed to alveolar pressure (or very nearly), whereas others are outside the influence of alveolar pressure but are very sensitive to the state of lung inflation. These two types of vessels are known as alveolar and extra-alveolar, respectively ( Fig. 4-7 ).

The alveolar vessels are largely capillaries that course through the alveolar walls. The pressure to which they are exposed is very nearly alveolar pressure. However, it can be shown that, when the lung is expanded from a very low lung volume, this pericapillary pressure falls below alveolar pressure because of surface tension effects in the alveolar lining layer. By contrast, during deflation from high lung volumes, the pericapillary pressure is very close to alveolar pressure.
The extra-alveolar vessels are not exposed to alveolar pressure. The caliber of these vessels is determined by the radial traction of the surrounding alveolar walls and therefore depends on lung volume. When the lung inflates, the caliber of these vessels increases; when the lung deflates, their caliber decreases because of the elastic tissue in their walls and also because of a small amount of smooth muscle tone. The important point is that extra-alveolar vessel resistance falls with lung inflation, whereas alveolar vessel (capillary) resistance rises with lung inflation.
The small vessels (of approximately 30 µm diameter) in the corners of the alveolar walls behave in a manner that is intermediate between that of the capillaries and the extra-alveolar vessels. These corner vessels can remain open when the capillaries are closed. Indeed, this is the normal appearance in zone 1 lung (see later section on the distribution of blood flow). However, the shape and attachments of the corner vessels are very different from those of the larger extra-alveolar vessels, and it is unlikely that the pressure outside them varies in the same way when the lung expands.
The extra-alveolar vessels are surrounded by a potential perivascular space, which has an important role in the transport of extravascular fluid in the lung. The lymph vessels run in this space, although lymph can also traverse the space outside lymph vessels. One of the earliest histologic signs of interstitial pulmonary edema is “cuffing” of the perivascular space around the extra-alveolar vessels. A distinction should be drawn between the net pressure (sum of forces per unit area) pulling on the wall of an extra-alveolar vessel on the one hand, and the fluid hydrostatic pressure in the perivascular space on the other. The fluid hydrostatic pressure determines the movement of fluid into this region, and there is evidence that this pressure is very low compared with the hydrostatic pressure in the interstitium of the alveolar wall. As a consequence, fluid that passes from the capillaries into the interstitial space of the alveolar wall eventually finds its way to the perivascular low-pressure region by virtue of the hydrostatic pressure gradient.
Pulmonary Vascular Resistance
Pulmonary vascular resistance is given by the following relationship:
Pulmonary arterial pressure − pulmonary venous pressure Pulmonary blood flow
It is important to appreciate that a single number for pulmonary vascular resistance is a very incomplete description of the pressure-flow properties of the pulmonary circulation. However, in practice, pulmonary vascular resistance is often a useful measurement because, although the normal value varies considerably, we often wish to compare the normal lung with a markedly abnormal one in which the vascular resistance is greatly increased.
In practice, pulmonary venous pressure is difficult to measure. Therefore the ratio of pulmonary artery pressure to blood flow is sometimes reported and is termed total pulmonary vascular resistance . An estimate of pulmonary venous pressure can be obtained by wedging a catheter in a small pulmonary artery (the so-called pulmonary wedge pressure).
Pressure-Flow Relations
If pulmonary blood flow is measured in an isolated, perfused lung, when pulmonary arterial pressure is raised (while pulmonary venous pressure, alveolar pressure, and lung volume are held constant), then flow increases relatively more than pressure ( Fig. 4-8 ). This figure shows that pulmonary vascular resistance decreases both when pulmonary arterial pressure is raised and when pulmonary venous pressure is raised (other pressures held constant) even as flow now falls. The unifying explanation for reduced resistance in both cases is vascular distention and recruitment due to raised intravascular pressure.

The decreases in pulmonary vascular resistance shown in Figure 4-8 help to limit the work of the right heart under conditions of high pulmonary blood flow. For example, during exercise, both pulmonary arterial and venous pressures rise. Although the normal pulmonary vascular resistance is remarkably small (the normal 5 L/min pulmonary blood flow is associated with an arterial-venous pressure difference of only approximately 10 mm Hg), the resistance falls to even lower values when the pulmonary arterial and venous pressures rise, as during exercise.
Two mechanisms responsible for the fall in pulmonary vascular resistance are recruitment , that is, opening up of previously closed blood vessels, and distention , that is, increase in caliber of vessels. Figure 4-9A shows experimental data from rapidly frozen dog lung preparations, indicating the importance of recruitment as the pulmonary arterial pressure is raised from low values. Note that the number of open capillaries per millimeter of length of alveolar wall increased from approximately 25 to over 50 as pulmonary arterial pressure was raised from zero to almost 15 cm H 2 O. Figure 4-9B shows data on the importance of distention of pulmonary capillaries. Note that the mean width of the capillaries increased from approximately 3.5 to nearly 7 µm as the capillary pressure was increased to approximately 50 cm H 2 O. Beyond that, there was very little change.

The mechanism of recruitment of pulmonary capillaries is not fully understood. It has been suggested that, as the pulmonary arterial pressure is increased, the critical opening pressures of various arterioles are successively overcome. However, it has been shown that the red blood cell concentration, used as a measure of perfusion, varied within areas supplied by single arterioles indicating that capillaries, not arterioles, probably accounted for the heterogenous perfusion. This suggests that vessels are recruited at the capillary rather than the arterial level.
A possible mechanism of recruitment of pulmonary capillaries is based on the stochastic properties of a dense network of numerous interconnected capillary segments. It can be shown in such a model that, if each capillary segment requires a very small critical pressure before flow begins and the network contains a distribution of these critical pressures, capillaries can be recruited over a large range of arterial pressures. For example, in a network with as many elements as in the human pulmonary capillary bed, a critical pressure of the order of only 0.02 cm H 2 O for the individual segments could result in recruitment over a range of arterial pressures from 0 to 30 cm H 2 O. Such a very small critical pressure could result from the intrinsic flow properties of blood, especially when the diameter of the red cells equals that of the capillary lumen.
The mechanism of distention of pulmonary capillaries is apparently simply the bulging of the capillary wall as the transmural pressure of the capillaries is raised. As Figure 4-9B shows, the mean capillary diameter increases as capillary transmural pressure rises. Probably this behavior is caused by a change in shape of the capillaries rather than actual stretching of the capillary wall. There is evidence that the strength of the wall (at least on the thin side) comes from the type IV collagen in the basement membranes (see later), which has a high tensile strength and Young’s modulus (i.e., it is very stiff). It is unlikely that it stretches appreciably when the capillary transmural pressure rises to 30 cm H 2 O. However, surface tension forces and also longitudinal tension in the alveolar wall associated with lung inflation tend to flatten the capillaries at low capillary transmural pressures, and this means that their diameter can increase when capillary pressure rises. In photomicrographs of rapidly frozen lung preparations, pulmonary capillaries with very high intracapillary pressures show remarkable bulging.
Recruitment and distention also provide mechanisms for increasing both the surface area of the lung microvasculature in contact with alveolar gas and the red cell transit time through the microvasculature, which may facilitate gas exchange.
Effect of Lung Volume
Lung volume has an important influence on pulmonary vascular resistance. Figure 4-10 shows that, as lung volume is increased from very low values, vascular resistance first decreases and then increases. The lung normally operates near the minimal value of vascular resistance, that is, FRC coincides with a low vascular resistance.

The increase in pulmonary vascular resistance at very low lung volume is caused by the decrease in caliber of the extra-alveolar vessels. Because these vessels are normally held open by the radial traction of the surrounding parenchyma, their caliber is least in the collapsed lung. Under these conditions the presence of elastic tissue and smooth muscle with tone in the wall of these vessels may result in a critical opening pressure of approximately 7 cm H 2 O. Also, at low lung volumes, vascular resistance is extremely sensitive to vasoconstrictor drugs, such as serotonin, which cause contraction of vascular smooth muscle.
Another factor that may contribute to the high pulmonary vascular resistance at low states of lung inflation is folding and distortion of pulmonary capillaries. However, the possible importance of distortion of the pulmonary capillaries as a cause of the increase of vascular resistance at low lung volumes is still uncertain.
At high states of lung inflation, the increase in pulmonary vascular resistance is probably caused by narrowing of the pulmonary capillaries. An analogy is a piece of thin rubber tubing that narrows considerably when it is stretched across its diameter. This distortion increases the resistance to fluid moving through it. Direct measurements on rapidly frozen dog lungs show that the mean width of the capillaries is greatly decreased at high states of lung inflation.
In considering the effects of lung inflation, a distinction should be made between “positive” and “negative” pressure inflation. The results shown in Figure 4-10 were found with negative-pressure inflation, that is, when the lung was expanded by reducing pleural pressure and the relationship between pulmonary arterial and alveolar pressures was held constant. If positive-pressure inflation is used (i.e., alveolar pressure is increased with respect to pulmonary arterial pressure), pulmonary vascular resistance increases even more at high states of lung inflation. The reason is that lung inflation is then associated with a decrease in the transmural pressure of the capillaries and they are, in effect, squashed by the increased alveolar pressure. This is actually the case in normal subjects, for example, during inhalation to total lung capacity. Although alveolar pressure remains at atmospheric pressure at the end of inspiration (glottis open), pulmonary arterial and venous pressures fall along with intrapleural pressure. Thus the net result is to decrease the transmural pressure across the pulmonary capillaries, and this is an additional contributing factor in the increase of pulmonary vascular resistance.
Other Factors Affecting Pulmonary Vascular Resistance
Various drugs affect pulmonary vascular resistance. In some instances, the effects depend on the species of animal. However, in general, serotonin, histamine, and norepinephrine cause contraction of pulmonary vascular smooth muscle and increase vascular resistance. These drugs are particularly effective as vasoconstrictors when the lung volume is small and the radial traction of surrounding parenchyma on the extra-alveolar vessels is weak. Drugs that often relax smooth muscle in the pulmonary circulation include acetylcholine and isoproterenol. However, normal pulmonary blood vessels have little resting tone, so the degree of potential relaxation is small.
The autonomic nervous system exercises a weak control on the pulmonary circulation. There is evidence that increased sympathetic tone can cause vasoconstriction and stiffening of the walls of the larger pulmonary arteries. Both α- and β-adrenergic receptors are present. Increased parasympathetic activity has a weak vasodilator action. As already indicated, any changes of vascular smooth muscle tone are much more effective at low states of lung inflation (when the extra-alveolar vessels are narrowed) or in the fetal state (when the amount of smooth muscle present is much greater than in the adult).
Pulmonary edema increases vascular resistance by a mechanism that is poorly understood. It may be that there are different mechanisms, depending on the type and stage of edema. Interstitial pulmonary edema causes marked cuffing of the perivascular spaces of the extra-alveolar vessels. Presumably this increases their vascular resistance, because, as already indicated, these vessels rely on the radial traction of the surrounding parenchyma to hold them expanded. In addition, however, it may be that edema in the interstitium of the alveolar wall encroaches on the pulmonary capillaries to some extent, thus increasing their vascular resistance. Pulmonary edema results in reduced ventilation of the most affected regions. This (see gas exchange section) reduces their local alveolar P o 2 , and this in turn may stimulate what is known as hypoxic pulmonary vasoconstriction. This is discussed in a later section and in Chapter 6 .
Distribution of Pulmonary Blood Flow
Just as for ventilation, blood flow is not partitioned equally to all alveoli, even in the normal lung. Both gravitational (topographic) and nongravitational factors affect the distribution of blood flow.
Normal Distribution
The topographic distribution of pulmonary blood flow can conveniently be measured using radioactive materials. In one technique, 133m Xe is dissolved in saline and injected into a peripheral vein. When the xenon reaches the pulmonary capillaries, it evolves into the alveolar gas because of its low blood solubility. The resulting distribution of radioactivity within the lung can be measured using a gamma camera or similar device and reflects the regional distribution of blood flow. Subsequently the distribution of alveolar volume is obtained by having the subject rebreathe radioactive xenon to equilibrium. By combining the two measurements, the blood flow per unit alveolar volume of the lung can be obtained. The distribution of blood flow can also be measured with radioactive albumin macroaggregates and with a variety of other radioactive gases, including 15 O-labeled carbon dioxide and 13 N. Functional magnetic resonance imaging of the lung has been used to assess distribution of pulmonary blood flow. This noninvasive technique does not expose subjects to radioactivity; therefore it can be used repetitively and shows great promise for the future.
In the normal upright human lung, pulmonary blood flow decreases approximately linearly with distance up the lung, reaching very low values at the apex. However, if the subject lies supine, apical and basal blood flow become the same, and now blood flow is less in the anterior (uppermost) than posterior (lowermost) regions of the lung. Thus blood flow distribution is highly dependent on gravitational effects. During exercise in the upright position, both apical and basal blood flow rates increase and the relative differences are reduced.
The factors responsible for the uneven topographic distribution of blood flow can be studied conveniently in isolated lung preparations. These studies show that, in the presence of normal vascular pressures, blood flow decreases approximately linearly up the lung as it does in intact humans. However, if the pulmonary arterial pressure is reduced, blood flows only up to the level at which pulmonary arterial equals alveolar pressures; above this point, no flow can be detected. If venous pressure is raised, the distribution of blood flow may become more uniform in the region of the lung below the point at which pulmonary venous equals alveolar pressure.
Three-Zone Model for the Distribution of Blood Flow
Figure 4-11 shows a simple model for understanding the factors responsible for the topographic inequality of blood flow in the lung. The lung is divided into three zones according to the relative magnitudes of the pulmonary arterial, alveolar, and venous pressures.

Zone 1 is that region of the lung above the level at which pulmonary arterial equals alveolar pressures; in other words, in this region, alveolar pressure exceeds arterial pressure. Measurements in isolated lungs show that there is no blood flow in zone 1, the explanation being that the collapsible capillaries close because the pressure outside exceeds the pressure inside. Micrographs of rapidly frozen lung from zone 1 show that the capillaries have collapsed, although occasionally trapped red blood cells can be seen within them.
The vertical level of blood flow can be influenced by the surface tension of the alveolar lining layer, as discussed earlier. If measurements are made on a lung immediately after it is inflated from a near-collapsed state, blood flow reaches 3 or 4 cm above the level at which pulmonary arterial and alveolar pressures are equal. This can be explained by the reduced surface tension, which lowers the pericapillary hydrostatic pressure.
Zone 2 is that part of the lung in which pulmonary arterial pressure exceeds alveolar pressure, but alveolar pressure exceeds venous pressure. Here the vessels behave like Starling resistors, that is, as collapsible tubes surrounded by a pressure chamber. Under these conditions, flow is determined by the difference between arterial and alveolar pressures, rather than by the expected arterial-venous pressure difference. One way of looking at this is that the thin wall of the vessel offers no resistance to the collapsing pressure, so the pressure inside the tube at the downstream end is equal to chamber pressure. Thus the pressure difference responsible for flow is perfusion minus chamber pressure. This behavior has been variously referred to as the waterfall or sluice effect and can be demonstrated in rubber-tube models on the laboratory bench. The increase in blood flow down zone 2 can be explained by the hydrostatic increase in pulmonary arterial pressure down the zone, whereas the alveolar pressure remains constant. Thus the pressure difference determining flow increases linearly with distance.
Zone 3 is that part of the lung in which venous pressure exceeds alveolar pressure. Radioactive gas measurements show that blood flow increases as one measures vertically down this zone, although, in some preparations at least, the rate of increase is apparently less than found in zone 2. Because the pressure difference responsible for flow is arterial minus venous pressure and because these two pressures increase similarly with distance down the zone, the increase in blood flow is not explained by changes in perfusing pressure. Instead, blood flow increases down this zone because vascular resistance falls with distance down the zone, likely because of progressive distention (confirmed histologically ) from the increasing transmural pressure (intravascular pressure increasing down the zone while alveolar pressure is constant). However, resistance may also be reduced by recruitment of capillaries.
The Effect of Lung Volume on the Distribution of Blood Flow—Zone 4
In spite of its simplicity, the three-zone model of Figure 4-11 , based on the effects of pulmonary arterial, alveolar, and venous pressures, accounts for many of the distributions seen in the normal lung. However, other factors play a role; one of these is lung volume. For example, under most circumstances, a zone of reduced blood flow, known as zone 4, is seen in the lowermost region of the upright human lung. This zone becomes smaller as lung volume is increased, but careful measurements indicate that a small area of reduced blood flow is present at total lung capacity at the lung base. As lung volume is reduced, this region of reduced blood flow extends further and further up the lung, so that at FRC, blood flow decreases in the bottom half of the lung. At RV the zone of reduced blood flow extends all the way up the lung, so that blood flow at the apex exceeds that at the base.
These patterns cannot be explained by the interactions of the pulmonary arterial, venous, and alveolar pressures as in Figure 4-11 . Instead, we have to take into account the contribution of the extra-alveolar vessels. As pointed out previously (see Fig. 4-10 ), the caliber of these vessels is determined by the degree of lung inflation; as lung volume is reduced, the vessels narrow. In the upright human lung, the alveoli are less well expanded at the base than at the apex because of distortion of the elastic lung caused by its weight (see Fig. 4-5 ). As a result the extra-alveolar vessels are relatively narrow at the base, and their increased contribution to pulmonary vascular resistance results in the presence of a zone of reduced blood flow in that region. As overall lung volume is reduced, the contribution of the extra-alveolar vessels to the distribution of blood flow increases, and zone 4 extends further up the lung. At residual volume the caliber of the extra-alveolar vessels is so small that they completely dominate the picture and determine the distribution of blood flow.
Vasoactive drugs and interstitial edema can modify the contribution of extra-alveolar vessels to pulmonary vascular resistance. For example, the role of the extra-alveolar vessels can be exaggerated by injecting vasoconstrictor drugs such as serotonin. Under these conditions, zone 4 extends even further up the lung. The opposite effect is seen if a vasodilator drug such as isoproterenol is infused into the pulmonary circulation. With interstitial edema, the contribution of the extra-alveolar vessels increases, because the edema creates a cuff of fluid around the vessels and thereby narrows them. This is thought to be the cause of the increased pulmonary vascular resistance seen at the base of the human lung in conditions of interstitial pulmonary edema, in which the distribution of blood flow often becomes inverted (e.g., in chronic mitral stenosis). Under these conditions the blood flow to the apex of the upright lung consistently exceeds the flow to the basal regions. However, the effects of interstitial edema on blood flow distribution are still not fully understood.
Other Factors Affecting the Distribution of Blood Flow
Because the topographic distribution of blood flow in the normal lung can be attributed to gravity, it is not surprising that, during increased acceleration, the distribution of blood flow becomes more uneven. For example, during exposure to +3 g acceleration, that is, three times the normal acceleration experienced by someone in the upright posture, the upper half of the lung is completely unperfused. The amount of unperfused lung is approximately proportional to the g level.
By contrast, in astronauts during sustained microgravity in space, the distribution of blood flow becomes more uniform. Because it is not possible to use radioactive gases in this environment, the inequality of blood flow has been determined indirectly from the size of the cardiogenic oscillations for P co 2 . Cardiogenic oscillations are fluctuations in the concentrations of gases such as oxygen, carbon dioxide, and nitrogen during a single expiration. They have the same frequency as heart rate and are considered to be caused by differential rates of emptying of different parts of the lung due to contraction and dilatation of the heart exerting direct pressure on nearby but not distant lung parenchyma. For oscillations to be detected, these differentially emptying regions must also have different alveolar P o 2 and P co 2 values, and this happens when blood flow and ventilation are not uniformly distributed throughout the lung. Microgravity almost abolishes cardiogenic oscillations, implying greater uniformity in the distribution of blood flow and/or ventilation in the absence of gravity. Interestingly, because these oscillations can still be seen, albeit to a much smaller extent than on earth, some inequality remains, indicating that gravity-independent mechanisms are also present.
Although gravity is a major factor determining the uneven distribution of blood flow in the upright human lung, it is now clear that nongravitational factors also play an important role. There are several possible mechanisms. One is that there may be regional differences of vascular conductance, with some regions of the pulmonary vasculature having an intrinsically higher vascular resistance than others. This has been shown to be the case in isolated dog lungs, and there is some evidence for higher blood flows in the dorsal-caudal than the ventral regions of the lung in both intact dogs and horses. Another possible factor is a difference in blood flow between the central and peripheral regions of the lung, although this finding is controversial. Some measurements show differences in blood flow along the acinus, with the more distal regions of the acinus being less well perfused than the proximal regions. Finally, as pointed out earlier, because of the complexity of the pulmonary circulation at the alveolar level, including the very large number of capillary segments, it is likely that there is inequality of blood flow at this level. Reference has already been made to the possibility of recruitment of pulmonary capillaries based on the stochastic properties of a dense network of numerous interconnected capillary segments. There is also work suggesting that the distribution of pulmonary blood flow in small vessels may follow a fractal pattern. The term fractal describes a branching pattern of both structure (blood vessels) and function (blood flow) that repeats itself with each generation. This means that any subsection of the vascular tree exhibits the same branching pattern as the entire tree. Were a picture of such a subsection to be enlarged, it would overlie and match the pattern of the whole tree. Just as mentioned for ventilation earlier, repeated branching of blood vessels has implications for how blood flow is distributed independently of gravitational influences. The greater the number of branch points, the greater the likely inequality of perfusion among alveoli. This implies that the finer the spatial resolution of the method used to assess flow distribution, the greater the amount of inequality likely to be detected.
Abnormal Patterns of Blood Flow
The normal distribution of pulmonary blood flow is frequently altered by lung and heart disease. Localized lung disease, such as fibrosis and cyst formation, usually causes a local reduction of flow. The same is true of pulmonary embolism, in which the local reduction in blood flow, as determined from a perfusion scan, is usually coupled with normal ventilation, and this pattern provides important diagnostic information. Bronchial carcinoma may reduce regional blood flow, and occasionally a small hilar lesion can cause a marked reduction of blood flow to one lung, presumably through compression of the main pulmonary artery. Generalized lung diseases, such as COPD and bronchial asthma, also frequently cause patchy inequality of blood flow. Sometimes, asthmatic patients whose disease is thought to be fairly well controlled show marked impairment of blood flow in some lung regions.
Heart disease frequently alters the distribution of blood flow, as might be expected from the factors responsible for the normal distribution (see Fig. 4-11 ). For example, patients with pulmonary hypertension or increased blood flow through left-to-right shunts usually show a more uniform distribution of blood flow. Diseases in which pulmonary arterial pressure is reduced, such as tetralogy of Fallot with oligemic lungs, are associated with reduced perfusion of the lung apices. Increased pulmonary venous pressure, as in mitral stenosis, initially causes a more uniform distribution than normal. However, in advanced disease, an inversion of the normal distribution of blood flow is frequently seen, with more perfusion to the upper than to the lower zones. The mechanism for this shift is not fully understood, but, as indicated earlier, perivascular edema causing an increased vascular resistance of the extra-alveolar vessels is thought to be a factor.
Active Control of the Pulmonary Circulation
The distribution of pulmonary blood flow and the pressure-flow relations of the pulmonary circulation are normally dominated by the passive effects of the hydrostatic pressure gradient described earlier. Thus the roles of gravity, of variation in vascular lengths and diameters, and of recruitment and distention can account for much of the behavior of the normal circulation. The normal adult pulmonary circulation has a limited amount of smooth muscle in the walls of the vessels, and active control of vascular tone is weak. However, in some conditions, there is an increase in the amount of smooth muscle. This is the case in the fetal lung, in long-term residence at high altitude, and in prolonged pulmonary hypertension. In these situations the tone of the vascular smooth muscle plays a more significant role. However, some active control of the circulation is seen in the normal lung.
Hypoxic Pulmonary Vasoconstriction
In a region of a lung with alveolar hypoxia, vascular smooth muscle contracts and raises local vascular resistance, which may reduce blood flow. The precise mechanism of such hypoxic pulmonary vasoconstriction is still not known, but, because it can be observed in excised isolated lungs, it clearly does not depend on central nervous system connections. Furthermore, excised segments of pulmonary artery can be shown to constrict if their environment is made hypoxic, so it appears to be a local action of the hypoxia on the artery itself. It is also known that it is the P o 2 of the alveolar gas, not of the pulmonary arterial blood, that chiefly determines the response. This can be proved by perfusing a lung with blood with a high P o 2 while keeping the alveolar P o 2 low; under these conditions the vasoconstrictive response is still seen.
The mechanisms of hypoxic pulmonary vasoconstriction are still not fully understood. Studies indicate that voltage-gated potassium channels in the smooth muscle cells are involved, leading to increased intracellular calcium ion concentrations. There may be roles for vasoactive substances generated by the endothelial cell. More details are covered in Chapter 6 .
Endothelium-derived vasoactive substances play a role. Nitric oxide (NO) is an endothelium-derived relaxing factor for blood vessels. It is formed from l -arginine and is a final common pathway for a variety of biologic processes. NO activates soluble guanylate cyclase, which leads to smooth muscle relaxation by the synthesis of cyclic guanosine monophosphate. Inhibitors of NO synthesis augment hypoxic pulmonary vasoconstriction in animal preparations, and inhaled NO reduces hypoxic pulmonary vasoconstriction in humans. The required inhaled concentration of NO is extremely low (approximately 20 parts per million), and the gas is very toxic at high concentrations. The effects of NO on ventilation-perfusion matching (see later) and arterial P o 2 in patients with lung disease depends on whether vasodilatation increases perfusion of well-ventilated regions of the lung or not.
Vasoconstrictor peptides, known as endothelins and released by pulmonary vascular endothelial cells, may participate as well. Their role in normal physiologic processes and disease is still being evaluated, but endothelin antagonists have become important therapeutic agents in pulmonary arterial hypertension.
The stimulus-response curve of hypoxic pulmonary vasoconstriction is very nonlinear ( Fig. 4-12 ). When the alveolar P o 2 is altered in the region above 100 mm Hg, little change in vascular resistance is seen. However, when the alveolar P o 2 is reduced to approximately 70 mm Hg, vasoconstriction begins, and, at a very low P o 2 approaching that of mixed venous blood, the local blood flow may be almost abolished. The data shown in Figure 4-12 are from anesthetized cats. However, there are species differences in the stimulus-response curves. For example, in the coatimundi (a small South American mammal), there is an almost linear reduction of blood flow between alveolar P o 2 values of 150 and 40 mm Hg. The preparation in which these measurements were made had the additional advantages that the chest was closed and the measurements were made in a very small region of lung. These conditions probably give the best information on the role of the phenomenon in the local regulation of blood flow.

The major site of the vasoconstriction is in the small pulmonary arteries. In the normal human lung the small arteries have a meager amount of smooth muscle, which may be uneven in its distribution. This may explain why, even in global alveolar hypoxia (e.g., at high altitude), vasoconstriction is nonetheless uneven. For example, alveolar hypoxia nearly doubles the dispersion of transit times through the pulmonary circulation of a lobe of dog lung, and the distribution of India ink particles injected into the pulmonary circulation during alveolar hypoxia is more uneven than during normoxia. This uneven vasoconstriction probably plays a role in the mechanism of high-altitude pulmonary edema (see later).
Hypoxic pulmonary vasoconstriction has the effect of directing blood flow away from hypoxic regions of lung, which is beneficial to gas exchange. Other things being equal, this reduces the amount of ventilation-perfusion inequality in a diseased lung and limits the depression of the arterial P o 2 . An example of this is seen in patients with asthma when they are treated with certain bronchodilators. These sometimes reduce arterial P o 2 as a result of an increase in blood flow to poorly ventilated areas. In patients with severe COPD with elevated pulmonary arterial pressure, prolonged nocturnal treatment with oxygen has been shown to reduce the degree of pulmonary hypertension and to improve the prognosis in these patients. The mechanism is presumably gradual inhibition by hyperoxia of increased smooth muscle tone originally caused by the hypoxia. The reduction in pulmonary hypertension in turn reduces afterload on the right ventricle.
Residence at high altitude results in hypoxic pulmonary vasoconstriction, both in newcomers and in permanent residents. The increase in pulmonary arterial pressure is especially marked during exercise. If 100% oxygen is given to normal subjects after they have been exposed to hypoxia for as little as 2 weeks, the pulmonary arterial pressure does not immediately return to the normal level. This suggests that hypoxia has already induced some structural change in the pulmonary vessels and new experiments show changes in ion channels controlling smooth muscle contraction in pulmonary vessels that depend on HIF-1a. There is considerable variation in the response of pulmonary arterial pressure to alveolar hypoxia, leading some investigators to divide people into “responders” and “nonresponders.”
Probably the most important role for hypoxic pulmonary vasoconstriction is in the perinatal period. During fetal life, when the lungs do not undertake gas exchange, pulmonary vascular resistance is very high, partly because of hypoxic vasoconstriction, and only some 15% of the cardiac output flows through the lungs. The rest bypasses the lungs via the ductus arteriosus. The vasoconstriction is particularly effective because of the abundance of smooth muscle in the pulmonary arteries. At birth, when the first few breaths oxygenate the alveoli, the vascular resistance falls dramatically because of relaxation of vascular smooth muscle, and pulmonary blood flow increases enormously. In this situation the release of hypoxic vasoconstriction is critical in the transition from placental to air breathing, and it is this situation that is presumably responsible for the evolutionary pressure to maintain the phenomenon.
Other Physiologic Substances Affecting the Pulmonary Circulation
Many peptides and other substances can potentially alter the tone of muscular pulmonary blood vessels, although the roles of these substances under physiologic conditions are still being clarified. They include angiotensin II, bradykinin, vasopressin, atrial natriuretic peptide, endothelin, somatostatin, products of both the cyclooxygenase and lipoxygenase arms of the arachidonic acid cascade, and calcitonin gene–related peptide. Some substances show species differences, and some evoke either vasoconstriction or vasodilation, depending on their concentration. Biogenic amines such as acetylcholine, histamine, serotonin, and norepinephrine also affect pulmonary vascular smooth muscle.
Damage to Pulmonary Capillaries by High Wall Stresses
The blood-gas barrier has a basic dilemma. On the one hand, the barrier has to be extremely thin to allow efficient gas exchange by passive diffusion.On the other hand, the blood-gas barrier must be immensely strong because of the large mechanical stresses that develop in the capillary wall when the pressure in the capillaries rises or when the wall is stretched by inflating the lung to high volumes. There is evidence that the blood-gas barrier is just strong enough to withstand the highest stresses to which it is normally subjected. Unusually high capillary pressures or lung volumes result in ultrastructural damage or “stress failure” of the capillary wall, leading to a high-permeability type of pulmonary edema, or even pulmonary hemorrhage.
When the capillary transmural pressure is raised in animal preparations, disruption of the capillary endothelium, alveolar epithelium, or sometimes all layers of the capillary wall is seen. In the rabbit lung the first changes are seen at a transmural pressure of approximately 24 mm Hg, and the frequency of breaks increases as the pressure is raised. Although at first sight these capillary pressures seem to be very high, there is now good evidence that the capillary pressure rises to the mid-30s (mm Hg) in the normal lung during heavy exercise. This is largely secondary to the increase in left ventricular filling pressure.
It can be shown that, at these increased capillary transmural pressures, the “hoop” or circumferential stresses in the capillary wall become extremely high. Indeed, they approach the breaking stress of collagen. The main reason for the very high stresses is the extreme thinness of the wall, which, in the human lung, is less than 0.3 µm in some places. It is now believed that the strength of the blood-gas barrier on the thin side comes from type IV collagen in the basement membranes. The thickness of the type IV collagen layer is only approximately 50 nm.
Stress failure is the mechanism of several clinical conditions characterized by high-permeability pulmonary edema or hemorrhage. Neurogenic pulmonary edema has been shown to be associated with very high capillary pressures, the edema is of the high-permeability type, and ultrastructural damage to the capillaries has been demonstrated, consistent with stress failure. High-altitude pulmonary edema is apparently caused by uneven hypoxic pulmonary vasoconstriction (referred to earlier), which allows some of the capillaries to be exposed to high pressure. Again, the edema is of the high-permeability type, and typical ultrastructural changes in the capillaries have been demonstrated in animal preparations.
A particularly interesting condition is seen in racehorses, which can suffer bleeding into the lungs while galloping. This is very common and is caused by the extremely high pulmonary capillary pressures, which approach 100 mm Hg. Direct evidence of stress failure of pulmonary capillaries has been shown in these animals. In fact, there is evidence that elite human athletes develop some ultrastructural changes in their blood-gas barrier during extreme exercise because significantly higher concentrations of red blood cells, total protein, and leukotriene B 4 are seen in their bronchoalveolar lavage fluid than in sedentary controls. This only happens at extremely high levels of exercise. A similar group of athletes who exercised at submaximal levels for 1 hour showed no changes in the bronchoalveolar lavage fluid.
Overinflation of the lung is known to increase the permeability of pulmonary capillaries. Stress failure is apparently the mechanism because it has been shown that, for the same capillary transmural pressure, the frequency of capillary wall damage is greatly increased at high lung volumes. This is because some of the increased tension in the alveolar wall associated with lung inflation is transmitted to the capillary wall. This may be important in ventilator-induced lung injury. Finally, conditions in which the basement membrane of the capillary wall is damaged are associated with alveolar bleeding.
Nonrespiratory Functions of the Pulmonary Circulation
Although the primary purpose of the pulmonary circulation is to provide the lung with mixed venous blood so that oxygen can be added and carbon dioxide removed, the pulmonary circulation has other functions, particularly those of metabolism.
A number of vasoactive substances are metabolized by the lung. Because it is the only organ whose microcirculation receives the whole cardiac output, the lung is uniquely situated to modify blood-borne substances. Indeed, a substantial fraction of all the vascular endothelial cells in the body is located in the lung.
The only known example of biologic activation by passage through the pulmonary circulation is the conversion of the relatively inactive polypeptide angiotensin I to the potent vasoconstrictor angiotensin II. The latter is up to 50 times more active than its precursor but is unaffected by passage through the lung. The conversion of angiotensin I is catalyzed by an enzyme, angiotensin I–converting enzyme, which is located in small pits (caveolae intracellulares) in the surface of the capillary endothelial cells.
A number of vasoactive substances are completely or partially inactivated during passage through the lung. Bradykinin is largely inactivated (up to 80%), and the enzyme responsible is angiotensin I–converting enzyme. The lung is the major site of inactivation of serotonin (5-hydroxytryptamine), not by enzymatic degradation, but by an uptake and storage process. Some of the serotonin may be transferred to platelets in the lung or stored in some other way and released during anaphylaxis. The prostaglandins E 1 , E 2 , and F 2α are also inactivated in the lung. Norepinephrine is also taken up by the lung to some extent (up to 30%). Histamine appears not to be affected by the intact lung.
Some vasoactive materials pass through the lung without significant gain or loss of activity. These include epinephrine, prostaglandins A 1 and A 2 , angiotensin II, and vasopressin (also called antidiuretic hormone).
Several vasoactive substances are normally synthesized or stored within the lung but may be released into the circulation in pathologic conditions. For example, in anaphylaxis, or during an asthma attack, histamine, bradykinin, prostaglandins, and “slow-reacting substance” are discharged into the circulation. Other conditions in which the lung may release potent chemicals include pulmonary embolism (see Chapter 57 ) and alveolar hypoxia.
There is also evidence that the lung plays a role in the clotting mechanism of blood under normal and abnormal conditions. For example, in the interstitium, there are large numbers of mast cells containing heparin. In addition, the lung is able to secrete special immunoglobulins, particularly immunoglobulin A, in bronchial mucus, which contribute to its defenses against infection. The synthesis of phospholipids such as dipalmitoylphosphatidylcholine, a component of pulmonary surfactant (see Chapter 8 ), is an important function of alveolar type II cells that prevents lung collapse. Surfactant turnover is rapid, and, if the blood flow to a region of lung is obstructed (e.g., by an embolus), surfactant may become locally depleted with consequent atelectasis. Protein synthesis is also significant because collagen and elastin form the structural framework of the lung. Under abnormal conditions, proteases are apparently liberated from leukocytes or macrophages in the lung, causing breakdown of proteins and possibly emphysema (see Chapter 43 ). Another important activity is carbohydrate metabolism, especially the elaboration of the mucins and proteoglycans of bronchial mucus (see Chapter 10 ).
In addition to its metabolic functions, the lung has other functions apart from its primary gas-exchanging role. One is to act as a reservoir for blood. As stated previously, the lung has a remarkable ability to reduce its pulmonary vascular resistance through the mechanisms of recruitment and distention as vascular pressures are raised. The same mechanisms allow the lung to increase its blood volume with relatively small rises in pulmonary arterial or venous pressures. Such changes can be seen, for example, when a subject lies down after standing and blood drains from the legs into the lung.
Another function of the lung is to filter blood. Small intravascular thrombi are removed from the circulation before they can reach the brain or other vital organs. There is also evidence that many white blood cells are sequestered by the lung, although the significance of this is not clear.
Blood Flow
Blood flow is as important for gas exchange as is ventilation. This has not always been appreciated, partly because the process of ventilation is more obvious, especially in the dyspneic patient, and is more accessible to measurement. Much has been learned about the pulmonary circulation in the past few years, especially its metabolic functions. The anatomy and function of the pulmonary circulation is also described in Chapters 1 and 6 .
Pressures of the Pulmonary Circulation
The pressures in the pulmonary circulation are very low compared with those in the systemic circulation, and this feature is responsible for much of its special behavior. The normal pressures in the human pulmonary artery are typically approximately 25 mm Hg systolic, 8 mm Hg diastolic, and 15 mm Hg mean. Normal mean systemic arterial pressure is approximately 100 mm Hg, which is six times higher than that in the pulmonary circulation. The evolutionary force to keep the pressures in the pulmonary circulation so low is the mechanical vulnerability of the extremely thin blood-gas barrier. Higher pressures in the pulmonary capillaries would cause stress failure of the capillary wall.
Pressure Inside Blood Vessels
Because the pulmonary arterial pressure is so low, hydrostatic effects within the pulmonary circulation are very important. The adult upright human lung is some 30 cm high, giving a hydrostatic difference in pressure of 30 cm blood between the extreme apex and the base, which is equivalent to approximately 23 mm Hg. As a result, there are very substantial differences in flow within the small pulmonary arteries and the capillaries between the top and bottom of the upright lung. This topic is discussed further in the section on the distribution of pulmonary blood flow.
Various techniques have been used to determine the pattern of pressure drop along the pulmonary blood vessels. These include measurement of the transudation pressure on the pleural surface of isolated lung, measurement of the pressure transient resulting from the injection of a slug of low- or high-viscosity blood into the pulmonary artery, and direct puncture of different-sized vessels along with direct measurement of hydrostatic pressure. The direct puncture measurements indicate that much of the normal pressure drop in the pulmonary circulation probably takes place in the pulmonary capillaries, and that the mean capillary pressure is approximately halfway between that in the pulmonary artery and that in the pulmonary vein ( Fig. 4-6 ). This distribution of the pressure drop is consistent with the main function of the pulmonary circulation, which is to expose as large an area of blood as possible to the alveolar gas.

The distribution of pressure along the pulmonary blood vessels depends on lung volume. At low states of lung inflation, the resistance of the extra-alveolar vessels (see next section) increases, and more pressure drop then takes place across the pulmonary arteries and veins. By contrast, there is evidence that, at very high states of lung inflation, the resistance of the capillary bed is increased, and therefore there will be an additional pressure drop in the capillaries.
The pressures in the pulmonary circulation are highly pulsatile; indeed, if we take the normal systolic and diastolic pressures in the main pulmonary artery as 25 and 8 mm Hg, respectively, this represents a much greater proportional change than the systolic-diastolic difference in systemic arteries (120 and 80 mm Hg, respectively). There is good evidence that the pulsatility of pressure, and therefore flow, extends to the pulmonary capillaries.
Pressures Outside Blood Vessels
Some pulmonary blood vessels are exposed to alveolar pressure (or very nearly), whereas others are outside the influence of alveolar pressure but are very sensitive to the state of lung inflation. These two types of vessels are known as alveolar and extra-alveolar, respectively ( Fig. 4-7 ).

The alveolar vessels are largely capillaries that course through the alveolar walls. The pressure to which they are exposed is very nearly alveolar pressure. However, it can be shown that, when the lung is expanded from a very low lung volume, this pericapillary pressure falls below alveolar pressure because of surface tension effects in the alveolar lining layer. By contrast, during deflation from high lung volumes, the pericapillary pressure is very close to alveolar pressure.
The extra-alveolar vessels are not exposed to alveolar pressure. The caliber of these vessels is determined by the radial traction of the surrounding alveolar walls and therefore depends on lung volume. When the lung inflates, the caliber of these vessels increases; when the lung deflates, their caliber decreases because of the elastic tissue in their walls and also because of a small amount of smooth muscle tone. The important point is that extra-alveolar vessel resistance falls with lung inflation, whereas alveolar vessel (capillary) resistance rises with lung inflation.
The small vessels (of approximately 30 µm diameter) in the corners of the alveolar walls behave in a manner that is intermediate between that of the capillaries and the extra-alveolar vessels. These corner vessels can remain open when the capillaries are closed. Indeed, this is the normal appearance in zone 1 lung (see later section on the distribution of blood flow). However, the shape and attachments of the corner vessels are very different from those of the larger extra-alveolar vessels, and it is unlikely that the pressure outside them varies in the same way when the lung expands.
The extra-alveolar vessels are surrounded by a potential perivascular space, which has an important role in the transport of extravascular fluid in the lung. The lymph vessels run in this space, although lymph can also traverse the space outside lymph vessels. One of the earliest histologic signs of interstitial pulmonary edema is “cuffing” of the perivascular space around the extra-alveolar vessels. A distinction should be drawn between the net pressure (sum of forces per unit area) pulling on the wall of an extra-alveolar vessel on the one hand, and the fluid hydrostatic pressure in the perivascular space on the other. The fluid hydrostatic pressure determines the movement of fluid into this region, and there is evidence that this pressure is very low compared with the hydrostatic pressure in the interstitium of the alveolar wall. As a consequence, fluid that passes from the capillaries into the interstitial space of the alveolar wall eventually finds its way to the perivascular low-pressure region by virtue of the hydrostatic pressure gradient.
Pulmonary Vascular Resistance
Pulmonary vascular resistance is given by the following relationship:
Pulmonary arterial pressure − pulmonary venous pressure Pulmonary blood flow
It is important to appreciate that a single number for pulmonary vascular resistance is a very incomplete description of the pressure-flow properties of the pulmonary circulation. However, in practice, pulmonary vascular resistance is often a useful measurement because, although the normal value varies considerably, we often wish to compare the normal lung with a markedly abnormal one in which the vascular resistance is greatly increased.
In practice, pulmonary venous pressure is difficult to measure. Therefore the ratio of pulmonary artery pressure to blood flow is sometimes reported and is termed total pulmonary vascular resistance . An estimate of pulmonary venous pressure can be obtained by wedging a catheter in a small pulmonary artery (the so-called pulmonary wedge pressure).
Pressure-Flow Relations
If pulmonary blood flow is measured in an isolated, perfused lung, when pulmonary arterial pressure is raised (while pulmonary venous pressure, alveolar pressure, and lung volume are held constant), then flow increases relatively more than pressure ( Fig. 4-8 ). This figure shows that pulmonary vascular resistance decreases both when pulmonary arterial pressure is raised and when pulmonary venous pressure is raised (other pressures held constant) even as flow now falls. The unifying explanation for reduced resistance in both cases is vascular distention and recruitment due to raised intravascular pressure.
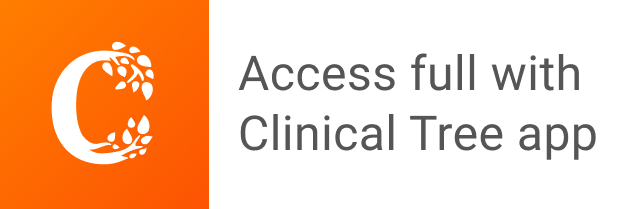