130 Autonomic abnormalities evidenced by sustained sympathetic overdrive and parasympathetic withdrawal are characteristic features of the heart failure (HF) state.1 This imbalance of autonomic activity has long been recognized as a cause of increased mortality and morbidity in patients with HF.2,3 In HF, sympathovagal imbalance leads to increased heart rate, excess release of proinflammatory cytokines, dysregulation of nitric oxide pathways, and arrhythmogenesis, all of which are adverse features that contribute directly or indirectly to worsening of HF and death. The increase of heart rate in patients with HF, for example, is a recognized predictor of mortality and morbidity,4 whereas the sustained increase of sympathetic activity contributes to progressive left ventricular (LV) dysfunction and LV remodeling.5 Pharmacologic interventions that reduce heart rate or limit sympathetic drive in patients with HF, such as β-blockers and ivabradine, a specific and selective inhibitor of the cardiac pacemaker current If, have been shown to improve survival, reduce hospitalization, and attenuate progressive LV remodeling.6,7 The past two decades have seen considerable emphasis placed on treating chronic HF through suppression of neurohumoral activation. This focus gave rise to beneficial drugs such as angiotensin-converting enzyme inhibitors, β-adrenergic receptor blockers, and aldosterone antagonists. More recently, renewed interest has emerged in modulating parasympathetic activity as a therapeutic target for treating chronic HF. Recognition that alteration in cardiac vagal efferent activity through peripheral cardiac nerve stimulation can produce bradycardia and can modify ventricular contractile function provided added support to this approach.8 Further support came from other investigations that uncovered the potential merits of electrical vagus nerve stimulation (VNS) in preventing sudden cardiac death, improving long-term survival, and suppressing ventricular arrhythmias.9,10 The theoretical and experimental foundations of VNS as a potential therapy for HF existed for decades, but only recently have advances in technology and instrumentation allowed for the development of implantable devices that can test the full range of safety and efficacy of this therapeutic approach in animal models of HF.11,12 One such device is the CardioFit VNS System (BioControl Medical, Yehud, Israel; Figure 130-1). The CardioFit VNS system delivers electrical stimulation via a lead placed surgically around the right cervical vagus nerve and attached to an implantable pulse generator.11 A second lead is placed in the right ventricle for electrocardiograph sensing and heart rate detection. Detection of heart rate is used to silence the VNS stimulator when heart rate drops below a preset level to avoid the adverse effects of bradycardia. Maximal stimulation current, pulse width, and operation algorithm of the CardioFit System are controlled by wireless communication and are used noninvasively to adjust the stimulation algorithm and settings for optimal outcome of therapy in each individual case. Figure 130-1 Right, Depiction of an implanted CardioFit Vagus Nerve Stimulation (VNS) device showing the position of the VNS lead on the right vagus nerve, the intracardiac pacing lead in the right ventricular apex, and the implantable CardioFit neurostimulator in the right subclavicular region. Left top, Depiction of the positioning of the CardioFit stimulation lead around the right vagus nerve. Left bottom, Photograph of the Cardiofit VNS implantable neurostimulator, sensing lead, and VNS lead. Courtesy BioControl Medical, Ltd., Yehud, Israel. In dogs with coronary microembolization-induced HF (LV ejection fraction of ~35%), long-term (3 months) VNS monotherapy was shown to improve LV ejection fraction, significantly reduce LV end-systolic and end-diastolic volumes, and increase stroke volume compared with untreated controls (Figure 130-2).11,13 The improvement of LV systolic function and prevention of progressive LV dilation was associated with a significant reduction of plasma levels of n-terminal-pro brain natriuretic peptide compared with controls (see Figure 130-2). Furthermore, ambulatory electrocardiograph Holter monitoring showed a reduction of minimum, average, and maximum heart rate by 1, 10, and 28 beats/min, respectively, in VNS-treated dogs compared to changes of heart rate in control dogs of only 2, 1, and 0.5 beats/min, respectively.11,13 Long-term VNS in dogs with HF also elicited improvements in indices of LV diastolic function evidenced by a decrease of LV end-diastolic pressure, increased deceleration time of rapid mitral inflow velocity, reduced LV end-diastolic circumferential wall stress, and increased the ratio of peak mitral inflow velocity in early diastole (PE) to peak mitral inflow velocity during left atrial contraction (PA) (see Figure 130-2).11,13 Because heart rate and LV wall stress decreased and because both are primary determinants of myocardial oxygen consumption (MVO2), it is reasonable to conclude that VNS can improve LV function without increasing MVO2, the latter being a desirable feature of therapies that target the HF state. Because VNS and β-blockade both reduce heart rate, VNS stimulation in dogs with HF was also examined on top of background therapy with β-blockade.14 In dogs with HF receiving background therapy with metoprolol succinate, the addition of VNS increased LV ejection fraction and decreased LV end-systolic volume compared to dogs treated with metoprolol alone.14 Figure 130-2 Left Top, Change Δ (treatment effect) between pretreatment and posttreatment of left ventricular (LV) end-diastolic volume (EDV), end-systolic volume (ESV), ejection fraction (EF), and stroke volume (SV) in untreated control dogs (blue bars) and dogs treated for 3 months with vagus nerve stimulation (VNS). *P < .05 versus control. Bottom left, Treatment effect, Δ, LV end-diastolic pressure (EDP), deceleration time (DT), end-diastolic wall stress (EDWS), and peak mitral valve inflow velocity ratio (PE/PA) in control dogs (blue bars) and dogs treated for 3 months with VNS. *P < .05 versus control. Right, Plasma levels of n-terminal pro-brain natriuretic peptide (nt-pro BNP) measured before initiating therapy (pretreatment) and 3 months after initiating therapy (posttreatment) in untreated control dogs and dogs treated for 3 months with VNS. VNS as a therapy for chronic HF was also examined in dogs with HF produced by high-rate ventricular pacing using the Cyberonics VNS system (Cyberonics, Houston, Texas).12 Dogs were randomized to control (n = 7) or to monotherapy with VNS (n = 8) and followed for 8 weeks with measurements made approximately 15 min after temporarily turning off the ventricular pacemaker. In these studies, VNS also resulted in a significant reduction of LV end-diastolic and end-systolic volumes and an increase of LV ejection fraction compared with controls.12 The improvement was accompanied by reduced plasma levels of norepinephrine, angiotensin-II, and C-reactive protein and increased baroreflex sensitivity. Because rapid pacing was maintained throughout the study except for short periods of time when measurements were made, one can argue that the benefits of VNS therapy, in this model of HF, are independent of heart rate.12 Several studies in dogs with microembolization-induced HF in which VNS was delivered long-term at stimulation levels that did not acutely decrease heart rate also showed significant improvements of LV ejection fraction along with reduction of LV size.15 These studies also showed that improvement of LV function with VNS was also associated with increased protein expression of SECA-2a and reduced expression of the proapoptotic protein caspase-3 in LV myocardium.16 In addition to preventing progressive global LV remodeling, long-term VNS was also shown to have an important beneficial effect on LV structural remodeling at the cellular level. In dogs with coronary microembolization-induced HF, VNS decreased the volume fraction of replacement fibrosis and the volume fraction of interstitial fibrosis.17 This finding suggests that VNS in HF can limit ongoing loss of functional cardiac units and can limit the accumulation of collagen in the cardiac interstitium and the expansion of the extracellular space, both of which favor improved LV systolic and diastolic function.17 In addition, VNS was shown to decrease oxygen diffusion distance and decrease cardiomyocyte hypertrophy.17 These alterations at the structural-cellular level can help to minimize the adverse effects of hypoxia and the burden of pathologic hypertrophy on the failing myocardium. Amelioration of these structural measures by VNS suggests that this form of therapy can also help preserve myocardial structural integrity. Vagal stimulation can activate multiple signaling pathways that act in concert to improve LV function in HF and suppress malignant ventricular arrhythmias. These include activation of muscarinic M2 and M3 receptors, inhibition of proinflammatory cytokines and normalization of nitric oxide signaling pathways (Figure 130-3). Figure 130-3 Diagram depicting potential mechanisms by which vagus nerve stimulation (VNS) elicits improvements of LV function and prevents development of malignant ventricular arrhythmias. Ach, Acetylcholine; M2, muscarinic M2 receptors; M3, muscarinic M3 receptors; HR, heart rate; MVO
Vagal Stimulation for Heart Failure
Effects of Vagus Nerve Stimulation on Left Ventricular Function and Chamber Remodeling in Experimental Heart Failure
Mechanisms That Underlie the Benefits of Vagus Nerve Stimulation in Heart Failure
Stay updated, free articles. Join our Telegram channel
Full access? Get Clinical Tree
Vagal Stimulation for Heart Failure
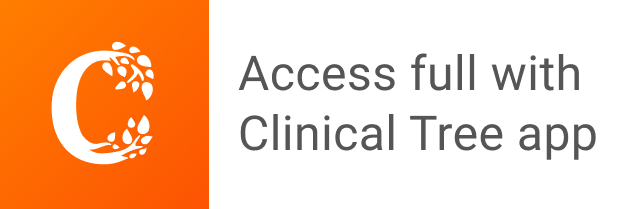