Introduction
Disorders of the soft tissue and bony structures of the chest wall generally cause restrictive dysfunction by limiting motion or causing disordered movement of the chest wall. Kyphoscoliosis and ankylosing spondylitis are disorders that involve the spine and its articulations, pectus excavatum involves the sternum, flail chest affects the ribs, and obesity adds to the soft tissues of the rib cage and abdomen. Kyphoscoliosis has the most severe impact on respiratory function and pectus excavatum the least. Alterations in respiratory mechanics produced by these disorders are discussed in concert with the most recent developments in diagnosis and treatment. Neuromuscular diseases that affect the chest wall are reviewed in Chapter 97 .
Kyphoscoliosis
Diagnosis and Etiology
The term kyphoscoliosis is derived from the Greek words kuphos and scolios (“humpback” and “crooked”) and refers to a group of disorders characterized by excessive spinal curvature in the lateral plane (scoliosis) and sagittal plane (kyphosis) as well as rotation of the spinal axis ( Fig. 98-1 ). Scoliosis, which is defined as a lateral spinal curvature greater than 10 degrees, is generally associated with kyphosis and rotation of the spine around its long axis. First described in detail by Hippocrates, kyphoscoliosis is one of the most common abnormalities of the spine, with an estimated prevalence for mild deformity of 1 in 1000 people and for severe deformity of 1 in 10,000 people in the United States.

Kyphoscoliosis is classified as idiopathic (no known cause), secondary or paralytic (associated with neuromuscular disease), and congenital (associated with vertebral malformation present at birth) ( Table 98-1 ). Idiopathic kyphoscoliosis, the most common form, usually manifests in late childhood or early adolescence and involves females more often than males with a ratio of 4 : 1. Idiopathic kyphoscoliosis is often seen in multiple members of one family. It is believed to be a multigene condition, with autosomal or sex-linked inheritance with variable phenotypic expression. A defect in the chromatin-remodeling gene family, CHD7, and several other genetic loci have been associated with susceptibility to idiopathic kyphoscoliosis. The most important sequelae of progressive kyphoscoliosis are back pain, psychosocial problems, and development of respiratory failure.
IDIOPATHIC |
Multigene disorder, developing by childhood/adolescence |
PARALYTIC OR SECONDARY |
|
CONGENITAL |
Due to spinal/vertebral malformations at birth |
The diagnosis of kyphoscoliosis is made by examining the chest wall. In severe cases, typical physical findings are the dorsal hump, which is due to angulated ribs and shoulder asymmetry, and the hip tilt, which is related to spinal rotation. In mild cases, the physical findings are more subtle. In children and adolescents, it is important to inspect the spine for asymmetry and to perform the Adam forward bend test. With this test, the examiner inspects the spine for thoracic or lumbar asymmetry while the patient bends forward at the waist until the spine becomes parallel to the floor. The Adam forward bend test can be used by community programs to screen for idiopathic kyphoscoliosis. When kyphoscoliosis is severe and complicated by right heart failure, clinical examination may reveal cyanosis, distended neck veins, peripheral edema, and hepatomegaly.
The diagnosis of kyphoscoliosis is confirmed by obtaining radiographs of the chest or spine. The severity of the spinal deformity can be assessed by measuring the Cobb angle of the vertebrae located within the spinal curvature. The Cobb angle is formed by the intersection of two lines, one parallel to the top and the other parallel to the bottom vertebrae of the scoliotic or kyphotic curves ( Fig. 98-2 ). The greater the Cobb angle, the more severe the deformity. Angles greater than 100 degrees are usually associated with respiratory symptoms such as dyspnea on exertion, and angles greater than 120 degrees with respiratory failure.

Pathophysiology
Pulmonary Function and Respiratory Mechanics
Kyphoscoliosis can produce the most severe restrictive impairment of all chest wall diseases ( Table 98-2 ). A number of factors contribute to the severity of the restrictive impairment. With idiopathic kyphoscoliosis, restrictive lung physiology is invariably present when the Cobb angle is greater than 90 degrees. With such severe deformity, total lung capacity (TLC) and vital capacity (VC) may be reduced to as low as 30% of predicted values. In contrast, residual volume (RV) may be normal or slightly increased, resulting in an elevated RV/TLC ratio. In children with severe spinal deformity, hypoplastic lung may contribute to reduced lung volumes. Other factors contributing to the restrictive process include the number of vertebrae involved, thoracic location of the curve, patient age, presence of kyphosis, degree of spinal rotation, and presence of weak respiratory muscles.
Parameter | KS | Post-Thor | PE | AS |
---|---|---|---|---|
TLC (% pred) | 45 | 65 | 90 | 85 |
VC (% pred) | 30 | 50 | 90 | 80 |
RV (% pred) | 95 | 90 | 100 | 100 |
FEV 1 (% pred) | 40 | 40 | 95 | 80 |
FEV 1 /FVC | 80 | 60 | 80 | 75 |
C rs (% pred) | 50 | 50 | — | 70 |
C cw (% pred) | 30 | 40 | — | 60 |
C l (% pred) | 60 | 50 | 80 | 80 |
P i max cm H 2 O | 40 | 50 | 90 | 80 |
MVV L/min | 37 | 37 | 107 | 80 |
The etiology of the kyphoscoliosis also influences the degree of respiratory impairment for any given Cobb angle. For example, in idiopathic kyphoscoliosis, those with Cobb angles less than 50 degrees usually have normal respiratory muscle strength whereas those with angles greater than 50 degrees may show mild to moderate reductions in maximal inspiratory pressure (P i max ) and maximal expiratory pressure (P e max ) due to either respiratory muscle weakness or altered mechanical advantage of the muscles. In comparison, individuals with secondary (paralytic) kyphoscoliosis also have primary respiratory muscle weakness and therefore may have a profound degree of pulmonary restriction, even when the Cobb angle is less than 50 degrees. In general, perhaps because of this primary involvement of the respiratory muscles, individuals with paralytic kyphoscoliosis have a greater restrictive defect for a given degree of spinal deformity than those with idiopathic kyphoscoliosis. Likewise, compared to those with idiopathic kyphoscoliosis, individuals with congenital kyphoscoliosis tend to have a greater restrictive impairment for a comparable degree of spinal deformity. In general, in congenital kyphoscoliosis, the loss in VC is 15% greater than in patients with idiopathic kyphoscoliosis and a similar Cobb angle, most likely due to associated rib deformities and underlying lung abnormalities.
Respiratory system compliance is reduced in kyphoscoliosis of any etiology, primarily due to the reduction in chest wall compliance and, to a lesser degree, a reduction in lung compliance due to microatelectasis. Cobb angles up to 50 degrees have minimal impact on respiratory compliance, whereas angles greater than 100 degrees may reduce compliance to levels seen in the adult respiratory distress syndrome. The stiff chest wall reduces the functional residual capacity (FRC). These factors combine to move the tidal breathing to a flatter, stiffer portion of the respiratory volume-pressure curve. This requires greater inspiratory effort for relatively small tidal breaths, which increases the work and oxygen cost of breathing ( Fig. 98-3 ). The increase in the oxygen cost of breathing may reach values three to five times those measured in healthy individuals and thus place patients at risk for respiratory muscle fatigue.

Exercise Capacity
Those with mild idiopathic kyphoscoliosis usually have normal exercise capacity. If exercise is limited, it is typically due to deconditioning rather than diminished ventilatory reserve. In contrast, individuals with moderate idiopathic kyphoscoliosis (Cobb angles 25 to 70 degrees), moderately severe kyphoscoliosis (Cobb angles 70 to 100 degrees), or severe kyphoscoliosis (Cobb angles greater than 100 degrees) may have exercise limitation due to ventilatory constraints. With severe deformities (Cobb angles greater than 100 degrees), cardiovascular factors may further contribute to exercise limitation. Supplemental O 2 may improve oxygenation during exercise but usually does not affect walk distance.
Control of Breathing and Sleep-Disordered Breathing
The disordered chest wall geometry in patients with kyphoscoliosis constitutes an elastic load placed on the inspiratory muscles. To compensate for this increased load, these individuals adopt a rapid shallow breathing pattern consisting of low tidal volumes (V t ) and shortened inspiratory time (T i ). This breathing pattern reduces the pressure needed to inhale (Pbreath) and minimizes the work per breath, thereby lowering the risk for developing respiratory muscle fatigue. Both parameters of rapid shallow breathing, V t and T i , decrease as the Cobb angle increases. However, rapid shallow breathing has the negative consequence of increasing dead space ventilation and promoting microatelectasis. As an additional compensatory mechanism, patients with increased elastic load also increase neural drive to the respiratory muscles. Indirect evidence for this compensatory strategy is the elevated mouth occlusion pressure at 100 ms (P 0.1 ) seen during quiet breathing, exercise, or breathing stimulated by hypercapnia. As the degree of kyphoscoliosis increases, the neural output to the respiratory muscles increases. That is, P 0.1 correlates positively with the Cobb angle in these patients. The increase in neural drive does not necessarily translate into increased minute ventilation because of the mechanical constraints imposed by the stiffened chest wall.
Hypoventilation is the most common sleep-related breathing disorder affecting individuals with kyphoscoliosis ( Fig. 98-4 ). Hypoventilation results in hypercapnia and hypoxemia and is most severe during REM sleep when there is a reduction in neural drive to the respiratory muscles. Nocturnal hypoxemia typically predates the onset of respiratory failure in these patients and significantly impacts the clinical course and quality of life of patients with kyphoscoliosis. Adverse sequelae of nocturnal hypoxemia and hypercapnia include respiratory muscle dysfunction, persistent hypercapnia and hypoxemia, pulmonary vascular remodeling, pulmonary hypertension, and ultimately cardiorespiratory failure and death. Of note, the Cobb angle does not correlate with the magnitude of nocturnal oxyhemoglobin desaturation. Accordingly, it is important to screen for nocturnal hypoventilation with overnight oximetry even in patients with only moderate spinal deformity. Obstructive sleep apnea may be seen with a frequency similar to that in the general population and, if present, can further aggravate nocturnal hypoventilation. Because sleep-related abnormalities and their effects on cardiorespiratory function are potentially treatable, they should always be evaluated in patients with kyphoscoliosis well in advance of development of daytime hypercapnia so that noninvasive ventilation can be instituted early.

Clinical Course
The etiology of kyphoscoliosis is a major determinant of the clinical course. Idiopathic kyphoscoliosis has the most benign clinical course. Secondary kyphoscoliosis may progress rapidly, largely dependent on the underlying cause of neuromuscular weakness and age of onset of the spinal deformity. An early age of onset with rapid curve progression during growth and progression after skeletal maturity increase one’s risk for serious respiratory complications. Congenital kyphoscoliosis may also progress rapidly.
In mild idiopathic kyphoscoliosis, the probability of developing respiratory failure with aging is similar to that in a healthy population. However, the risk is higher for patients with moderate or severe deformities. Large spinal curves at presentation, skeletal immaturity, and a thoracic, as opposed to a thoracolumbar or lumbar, location of the curve apex are generally considered risk factors for curve progression. The presence of respiratory muscle weakness enhances the likelihood of developing respiratory failure. In general, the spinal deformity worsens at a rate of about 1 degree per year in individuals with thoracic deformities greater than 50 degrees at skeletal maturity.
Individuals with severe idiopathic kyphoscoliosis and Cobb angles around 100 degrees should be monitored closely for respiratory complications, especially if they are middle-aged or older. Initially, individuals may experience dyspnea only with exertion but, as they age and the spinal deformity progresses, they may develop dyspnea even at rest. Cardiopulmonary problems and psychosocial concerns develop at a variable rate. When cor pulmonale develops, the prognosis is poor and, without therapy, death may supervene within one year. In addition to the degree of chest wall deformity, other factors that contribute to ventilatory failure in patients with idiopathic kyphoscoliosis are inspiratory muscle weakness, sleep-disordered breathing, and airway compression due to distortion of the lung parenchyma and twisting of airways. When respiratory failure develops in a patient with kyphoscoliosis and a Cobb angle less than 100 degrees, other causes for respiratory failure should be sought, particularly treatable causes such as sleep disordered breathing.
Treatment
Medical Treatment
General supportive measures include immunizations against influenza and pneumococci, smoking cessation, maintenance of a normal body weight, supplemental oxygen, and prompt treatment of respiratory infections. Physical activity should be encouraged to minimize deconditioning. Orthopedic braces have been used in skeletally immature patients with idiopathic kyphoscoliosis in an effort to prevent or correct the spinal deformity. A brace is generally recommended for growing children with angles between 25 and 40 degrees.
Noninvasive nocturnal ventilation constitutes a major advance in the treatment of individuals with severe kyphoscoliosis. Indications for instituting noninvasive nocturnal ventilation include symptoms suggestive of nocturnal hypoventilation or signs of cor pulmonale with either an elevated daytime arterial P co 2 or nocturnal oxygen saturation less than 88% for 5 consecutive minutes ( Table 98-3 ). Either pressure or volume preset ventilators are effective in providing noninvasive ventilation. Supplemental oxygen is indicated if hypoxemia persists despite correction of hypoventilation. For chronic respiratory failure, chest physiotherapy, bronchodilators, and diuretics may be needed in addition to noninvasive positive pressure ventilation. In those intolerant of noninvasive ventilation or unable to manage excessive bronchial secretions, tracheostomy constitutes another option.
|
The benefits of noninvasive ventilation are well established and include improvements in gas exchange, hemodynamic parameters, sleep architecture, quality of life, and perhaps survival ( Table 98-4 ). Long-term noninvasive ventilation has been shown to reduce the number and duration of hospitalizations and possibly increase survival, although this evidence is not yet supported by randomized controlled studies. Interestingly, noninvasive ventilation does not appear to improve respiratory muscle strength or endurance; instead, noninvasive ventilation may improve respiratory failure by enhancing central chemosensitivity to carbon dioxide.
GAS EXCHANGE |
|
Hemodynamics |
|
MECHANICS |
|
SLEEP HYGIENE |
|
OUTCOME |
|
Surgical Treatment
In general, surgery involves fusion of the affected vertebral bodies via the posterior approach and the use of devices such as rods, wires, hooks, and pedicle screws to support the spine. This approach, which has seen significant advances since the initial introduction of implantable Harrington rods in the 1960s, allows for multiplanar correction, stable fixation with less spinal fusion, and early postoperative mobilization without a cast or brace. Newer nonfusion techniques use growth-friendly rods such as expandable spinal rods, titanium rib implants, or remotely operated magnetic growth rods. Surgery is currently recommended in individuals with skeletal immaturity and a Cobb angle greater than 45 degrees.
The benefits of spinal surgery on pulmonary function remain unclear. Immediately following surgery, pulmonary function may be impaired due to rib cage trauma and changes in the chest wall caliber. In the long-term, pulmonary function is more likely to improve in young children and adolescents than in adults. In the absence of randomized controlled studies assessing the efficacy of surgery, the overall role of surgical management in restoring function and minimizing the possibility of respiratory failure is not clear.
Thoracoplasty
Before the advent of effective antituberculosis chemotherapy, thoracoplasty was commonly employed to reduce lung volume as a way to control pulmonary tuberculosis. Thoracoplasty reduced lung volume using a variety of means: removing or fracturing ribs ( Fig. 98-5 ), resecting the phrenic nerve, or filling the pleural space with foreign material (i.e., Lucite spheres [ Fig 98-6 ], oil [ Fig. 98-7 ], and ping pong balls). Severe restrictive impairment may manifest itself decades after the procedure; after several years without symptoms, individuals may become dyspneic, initially with exertion and then at rest, and may eventually develop chronic respiratory failure. Factors such as slowly progressive kyphoscoliosis, in part, account for the delay in the onset of symptoms. The severity of the restrictive defect, which can be similar to that seen in kyphoscoliosis (see Table 98-2 ), may be due to a reduction in respiratory system compliance, fibrothorax, lung resection, phrenic nerve damage, scoliosis, and lung fibrosis related to prior underlying granulomatous disease.



Since this procedure was abandoned for tuberculosis therapy after the 1950s, very few post-thoracoplasty patients are alive today. However, knowledge of the sequelae of thoracoplasty is of more than historical interest. Thoracoplasty is occasionally still performed to treat bronchopleural fistulas that have failed to close following decortication or persistent empyema in which decortication is not feasible or has failed to eradicate the infection. Surgery similar to thoracoplasty includes aggressive surgery on the rib cage, as may be performed to resect tumors. Such surgeries may stiffen the chest wall and lead to an increase in the work of breathing, impaired gas exchange, and ultimately cor pulmonale. As with severe kyphoscoliosis, the treatment consists of oxygen, antibiotics when appropriate, and noninvasive nocturnal mechanical ventilation. Patients undergoing extensive chest wall surgery of any type should have their pulmonary function monitored periodically.
Pectus Excavatum
Diagnosis and Etiology
Pectus excavatum is a common congenital chest wall deformity characterized by excessive depression of the sternum and its adjacent costal cartilages ( Fig. 98-8 ). It is the most common chest wall deformity, seen in approximately 0.5% to 2% of the population, more frequently in males than in females (ratio 4 : 1). Diagnosed by inspecting the rib cage, pectus may be apparent in infancy but, in most cases, becomes noticeable at puberty.

Pectus excavatum may result from abnormal cartilage growth displacing the sternum inward. A genetic predisposition is supported by a large study in which 43% of patients with this deformity gave a family history of pectus excavatum. Approximately 6% of patients have a connective tissue disorder such as Marfan or Ehlers-Danlos syndromes, and 40% to 60% have scoliosis. Congenital heart disease may coexist in roughly 2% of cases.
The magnitude of sternal deformity is best assessed by computed tomography (CT) of the chest. The transverse (Tr) and anteroposterior (AP) diameters of the rib cage are measured at the level of the deepest sternal depression. The normal Tr/AP ratio, also known as the Haller index, is 2.5 or less (see Fig. 98-8 ). A Haller index of greater than 3.25 indicates a significant pectus deformity that may need surgical correction. Individuals with a significant deformity commonly have cosmetic complaints and, in addition, 30% to 70% of individuals may complain of exercise limitation or dyspnea with exertion, often out of proportion to recognized abnormalities in cardiopulmonary function.
Pathophysiology
Respiratory Mechanics and Exercise Capacity
Most patients with pectus excavatum have normal pulmonary function with a normal mobility of the rib cage. Occasionally, patients will have a mild restrictive deficit, often when there is concomitant scoliosis. Cardiopulmonary exercise testing is usually normal, although those with the most severe deformities may exhibit a mild reduction in maximal work rate and oxygen consumption. Although the concept is not widely accepted, right ventricular compression by the depressed sternum ( Fig. 98-9 and ) may be a mechanism contributing to exercise limitation in some individuals.


Treatment
Surgical correction of pectus excavatum is usually considered for patients with a Haller index greater than 3.5 in conjunction with symptoms or evidence of cardiac or pulmonary impairment. The modified Ravitch procedure consists of resection of costal cartilage and a sternal osteotomy, with or without fixation of the sternum by external or internal supports. The less invasive Nuss procedure entails placing a curved metal rod under the sternum through small incisions on each side of the rib cage. The rod is rotated under the sternum forcing and holding it outward; the rod is removed after 2 to 4 years once the sternum is stabilized in its proper position. The rate of complications does not differ significantly between these surgical approaches. Another minimally invasive procedure under investigation uses magnets to pull out the sternum and correct the deformity gradually, in a fashion analogous to that of braces, which gradually correct the position of teeth. Complications of procedures that involve cartilage resection include sternal necrosis, infection, or recurrence of the deformity. With corrective procedures, improvement of symptoms and function is difficult to predict. To date, there is no convincing evidence that correction of the deformity improves either cardiopulmonary function or exercise capacity.
Flail Chest
Diagnosis and Etiology
Flail chest arises when a segment of the rib cage moves inward rather than outward during inspiration ( ). Production of a flail segment of chest wall requires multiple rib fractures, specifically double fractures of three or more contiguous ribs or combined sternal and rib fractures, to uncouple the segment from the surrounding chest wall. An unstable segment of the chest wall can also result from multiple single rib fractures along a straight line that partially uncouples the segment from the surrounding chest wall. This condition is referred to as “nonintegrated chest wall” rather than “flail chest.”
The most common cause of flail chest is blunt chest trauma. Flail chest is most commonly seen after automobile accidents and falls, although it may also develop after aggressive cardiopulmonary resuscitation or in patients with pathologic rib fractures. In children, flail chest is infrequent because the chest wall is more compliant; thus, when a child does have a flail chest, it signifies a much greater degree of trauma. Rarely, flail chest may complicate corrective rib resection or appear in neonatal life due to congenital rib abnormalities.
The diagnosis of flail chest is made by examining the rib cage and noting the characteristic paradoxical movement of a chest wall segment inward rather than outward during inspiration (and outward rather than inward during expiration) with spontaneous breathing ( Fig. 98-10 and see ). In subtle cases, the paradoxical motion of the flail chest can be appreciated by palpation of the chest wall. Chest radiographs may confirm the presence of multiple rib fractures; however a three-dimensional reconstruction of a chest CT is a more sensitive technique for identifying rib fractures and the other pulmonary injuries, such as pulmonary contusion or hemothorax related to chest wall trauma. In the mechanically ventilated trauma patient, the diagnosis of flail chest may be delayed for days or even weeks and become apparent only after withdrawal of sedation and resumption of spontaneous breathing.

Trauma and Flail Chest
The presence of flail chest following trauma signifies severe thoracic injury and is an independent risk factor for respiratory complications, respiratory failure, and death. Patients with flail chest may have pulmonary complications such as lung contusion, pneumothorax, or hemothorax. Flail chest and its associated pain promote atelectasis, impair cough, and may lead to respiratory failure. Patients with flail chest are twice as likely to develop respiratory failure and require mechanical ventilation as patients with isolated lung contusion.
The presence of flail chest is thus associated with a poor prognosis and should alert the clinician that there may be traumatic injury to other organs. The presence of flail chest is a marker of increased mortality both in patients with isolated chest wall trauma and in patients with multiple sites of trauma. Without flail chest, mortality from chest wall trauma ranges from 7% to 14%; with concomitant flail chest, mortality increases further. Similarly, in patients with multiple sites of trauma, mortality is roughly 30%; in those with concomitant flail chest, mortality may be as high as 68%. The high mortality associated with flail chest is in part due to coexistence of other injuries such as fractures of long bones or vertebrae, head trauma, rupture of major vascular structures, or laceration of abdominal organs. Concomitant head injury and age older than 65 years are particularly poor prognostic factors in patients with traumatic flail chest. In patients who survive the acute injury, chronic disability related to flail chest may develop, with chest tightness, chest pain, or dyspnea on exertion. However, with operative fixation of the flail segment even years after the injury, these symptoms can improve dramatically.
Pathophysiology
Respiratory Mechanics
Flail chest disrupts the anatomic and functional integrity of the chest wall and can seriously alter its function. With flail chest, a segment of the chest wall is uncoupled from the forces that expand the rib cage. Consequently, this uncoupled segment moves passively with the variations of pleural pressure. With inspiration and the decrease in pleural pressure, the flail segment moves inward and, during expiration with less negative pleural pressure, it moves outward. If the normal swings in pleural pressure are amplified by a decrease in lung compliance due to concomitant pulmonary contusion and/or atelectasis, the paradoxical motion of the flail segment can increase.
The location of the flail segment is dependent on the site of rib fractures. The most common location is the lateral rib cage. However, for any flail segment, one may see different patterns of movement. For example, motion may be paradoxical within the rib cage itself or between the rib cage and the abdomen (see Fig. 98-10 ). The degree of paradoxical motion may also differ at different locations. Posterior rib fractures exhibit less paradoxical motion due to splinting provided by the paraspinal muscles. Normal recruitment patterns of the intercostal muscles may be altered because of severe chest wall pain and may increase or decrease the degree of rib cage paradox. Different pain-induced respiratory muscle recruitment patterns may explain why some cases of flail chest remain unstable following prolonged mechanical ventilation and why lateral flail segments have a higher propensity for dislocation following surgical fixation.
Respiratory Failure
The pathogenesis of respiratory failure in flail chest is multifactorial; hypoventilation due to pain, flail-induced impairment in respiratory muscle function, and concomitant lung injury all play a role. The simplistic theory that respiratory failure resulted from the movement of air back and forth from the injured to uninjured side ( pendelluft ) has been abandoned. A flail segment has important physiologic consequences, even in the absence of contusion. First, pain impairs cough and promotes shallow tidal breathing, both of which may lead to atelectasis. Second, the flail segment impairs respiratory muscle performance. The paradoxical motion of the chest wall increases the degree of muscle shortening for a given tidal volume and, because the muscles are shortening further, the work they perform is increased per breath. Increased work of breathing, together with an inefficient use of respiratory muscles increase the oxygen cost of breathing. When these factors are compounded by increases in elastic loads related to lung contusion and/or atelectasis along with hypoxemia, the respiratory muscles are predisposed to fatigue and respiratory failure or weaning difficulties ( Fig. 98-11 ).

Pulmonary Function Tests
Forced vital capacity (FVC) and FRC are reduced immediately following trauma sufficient to cause flail chest. VC may be reduced to 50% of predicted. These reductions are related to pain, disordered chest wall motion, and underlying lung contusion. In patients with flail chest without lung contusion treated conservatively, FVC and FRC generally return to baseline values within 6 months of the acute injury. In contrast, in patients with flail chest complicated by lung contusion treated conservatively, pulmonary function may be reduced up to 4 years after injury, most likely due to fibrosis of the contused area. Compared with conservative management, operative fixation of flail chest may be a better option to preserve pulmonary function, as indicated from both controlled and uncontrolled studies.
Treatment
General Aspects
The restoration of anatomic and functional integrity of the chest wall is a key for avoiding complications related to flail chest. Surgical and nonsurgical approaches can be used to stabilize the flail segment ( Fig. 98-12 ). Nonsurgical management is based on administration of adequate analgesia, clearing of bronchial secretions, and mechanical ventilatory assistance if needed. Control of pain is crucial in averting atelectasis and achieving an effective cough. Adequate pain relief can be accomplished by oral medications, patient-controlled analgesia pumps, intercostal nerve blocks, or epidural anesthesia. Adequate analgesia in combination with supplemental oxygen, effective tracheobronchial toilet, and cautious fluid replacement, often results in successful treatment of flail chest and avoidance of respiratory failure. If needed, mechanical ventilation can act as a pneumatic splint to stabilize the flail segment by keeping pleural pressure positive. However, mechanical ventilation delivered via a tracheostomy or endotracheal tube solely for the purpose of providing stability to the chest wall is not recommended due to the increased morbidity and mortality associated with mechanical ventilation. In contrast, positive pressure ventilation delivered noninvasively via a nasal or face mask to patients who are spontaneously breathing and able to protect their upper airway provides an alternate means of stabilizing the flail segment. Noninvasive ventilation, in conjunction with patient-controlled analgesia, improves gas exchange, allows for early patient mobilization and access for physiotherapy, and significantly reduces morbidity and mortality when compared with mechanical ventilation. If intubation and mechanical ventilation is needed, a low impedance ventilator mode that minimizes the likelihood of generating subatmospheric pleural pressure are optimal choices for stabilizing the flail segment and for weaning.
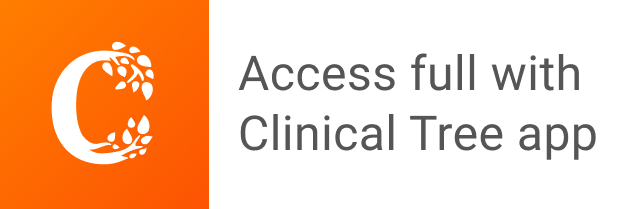