The repetitive, synchronized contraction and relaxation of the cardiac muscle cells provide the forces necessary to pump blood through the systemic and pulmonary circulations. In this chapter, we describe (1) basic mechanical features of this cardiac pump, (2) factors that influence and/or regulate the cardiac output, and (3) sources of energy and energy costs required for myocardial activity.
CARDIAC CYCLE
Left Pump
The mechanical function of the heart can be described by the pressure, volume, and flow changes that occur within it during a single cardiac cycle. A cardiac cycle is defined as one complete sequence of contraction and relaxation. The normal mechanical events of a cycle of the left heart pump are correlated in Figure 3–1. This important figure summarizes a great deal of information and should be studied carefully.
VENTRICULAR DIASTOLE
The diastolic phase1 of the cardiac cycle begins with the opening of the atrioventricular (AV) valves. As shown in Figure 3–1, the mitral valve passively opens when left ventricular pressure falls below left atrial pressure and the period of ventricle filling begins. Blood that had previously accumulated in the atrium behind the closed mitral valve empties rapidly into the ventricle, and this causes an initial drop in atrial pressure. Later, the pressures in both chambers slowly rise together as the atrium and ventricle continue passively filling in unison with blood returning to the heart through the veins.
Proper filling of the ventricles depends on three conditions: (1) the filling pressure of blood returning to the heart and atria, (2) the ability of the AV valves to open fully (not be stenotic), and (3) the ability of the ventricular wall to expand passively with little resistance (ie, to have high compliance). The healthy heart is very compliant during diastole so that filling normally occurs with only small increases in ventricular pressure.
Atrial contraction is initiated near the end of ventricular diastole by the depolarization of the atrial muscle cells, which causes the P wave of the electrocardiogram. As the atrial muscle cells develop tension and shorten, atrial pressure rises and an additional amount of blood is forced into the ventricle. At normal resting heart rates, atrial contraction is not essential for adequate ventricular filling. This is evident in Figure 3–1 from the fact that the ventricle has nearly reached its maximum or end-diastolic volume before atrial contraction begins. Atrial contraction plays an increasingly significant role in ventricular filling as heart rate increases because the time interval between beats for passive filling becomes progressively shorter with increased heart rate. Note that throughout diastole, atrial and ventricular pressures are nearly identical. This is because a normal open mitral valve has very little resistance to flow and thus only a very small atrial–ventricular pressure difference is necessary to produce ventricular filling.
Figure 3–1. Cardiac cycle—left heart pump. Cardiac cycle phases: A, diastole; B, systole that is divided into 3 periods; C, isovolumetric contraction; D, ejection; and E, isovolumetric relaxation.
VENTRICULAR SYSTOLE
Ventricular systole begins when the action potential passes through the AV node and sweeps over the ventricular muscle—an event heralded by the QRS complex of the electrocardiogram. Contraction of the ventricular muscle cells causes intraventricular pressure to rise above that in the atrium. Because of the valve structure, the increased pressure behind the leaflets in the ventricle causes abrupt closure of the AV valve.
Pressure in the left ventricle continues to rise sharply as the ventricular contraction intensifies. When the left ventricular pressure exceeds that in the aorta, the aortic valve passively opens. The period between mitral valve closure and aortic valve opening is referred to as the isovolumetric contraction phase because during this interval the ventricle is a closed chamber with a fixed volume. Ventricular ejection begins with the opening of the aortic valve. In early ejection, blood enters the aorta rapidly and causes the pressure there to rise. Pressure builds up simultaneously in both the ventricle and the aorta as the ventricular muscle cells continue to contract in early systole. This interval is often called the rapid ejection period.
Left ventricular and aortic pressures ultimately reach a maximum called peak systolic pressure. At this point, the strength of ventricular muscle contraction begins to wane. Muscle shortening and ejection continue, but at a reduced rate. Aortic pressure begins to fall because blood is leaving the aorta and large arteries faster than blood is entering from the left ventricle. Throughout ejection, very small pressure differences exist between the left ventricle and the aorta because the aortic valve orifice is so large that it presents very little resistance to flow.
Eventually, the strength of the ventricular contraction diminishes to the point where intraventricular pressure falls below aortic pressure. Because of the aortic valve structure, the increased pressure behind the leaflets in the aorta causes abrupt closure of the aortic valve. A dip, called the incisura or dicrotic notch, appears in the aortic pressure trace because a small volume of aortic blood must flow backward to fill the space behind the aortic valve leaflets as they close. After aortic valve closure, intraventricular pressure falls rapidly as the ventricular muscle relaxes. For a brief interval, called the isovolumetric relaxation phase, the mitral valve is also closed. Ultimately, intraventricular pressure falls below atrial pressure, the AV valve opens, and a new cardiac cycle begins.
Note that atrial pressure progressively rises during ventricular systole because blood continues to return to the heart and fill the atrium. The elevated atrial pressure at the end of systole promotes rapid ventricular filling once the AV valve opens to begin the next heart cycle.
The ventricle has reached its minimum or end-systolic volume at the time of aortic valve closure. The amount of blood ejected from the ventricle during a single beat, the stroke volume, is equal to ventricular end-diastolic volume minus ventricular end-systolic volume. Note that under normal conditions, the heart ejects only about 60% of its end-diastolic volume.
The aorta distends or balloons out during systole because more blood enters the aorta than leaves it. During diastole, the arterial pressure is maintained by the elastic recoil of walls of the aorta and other large arteries. Nonetheless, aortic pressure gradually falls during diastole as the aorta supplies blood to the systemic vascular beds. The lowest aortic pressure, reached at the end of diastole, is called diastolic pressure. The difference between diastolic and peak systolic pressures in the aorta is called the arterial pulse pressure. Typical values for systolic and diastolic pressures in the aorta are 120 and 80 mm Hg, respectively.
At a normal resting heart rate of approximately 70 beats/min, the heart spends approximately two-thirds of the cardiac cycle in diastole and one-third in systole. When increases in the heart rate occur, both diastolic and systolic intervals become shorter. Action potential durations are shortened and conduction velocity is increased. Contraction and relaxation rates are also enhanced. This shortening of the systolic interval tends to blunt the potential adverse effects of increases in the heart rate on diastolic filling time.
Right Pump
Because the entire heart is served by a single electrical excitation system, similar mechanical events occur essentially simultaneously in both the left and right sides of the heart. Both ventricles have synchronous systolic and diastolic periods, and the valves of the right and left sides of the heart normally open and close nearly in unison. Because the two sides of the heart are arranged in series in the circulation, they must pump the same amount of blood and therefore must have identical stroke volumes.
The major difference between the right and left pumps is in the magnitude of the peak systolic pressure. The pressures developed by the right side of the heart, as shown in Figure 3–2, are considerably lower than those for the left side of the heart (Figure 3–1). This is because the lungs provide considerably less resistance to blood flow than that offered collectively by the systemic organs. Therefore, less arterial pressure is required to drive the cardiac output through the lungs than through the systemic organs. Typical pulmonary artery systolic and diastolic pressures are 24 and 8 mm Hg, respectively.
Figure 3–2. Cardiac cycle—right heart pump.
The pressure pulsations that occur in the right atrium are transmitted in retrograde fashion to the large veins near the heart. These pulsations, shown on the atrial pressure trace in Figure 3–2, can be visualized in the neck over the jugular veins in a recumbent individual. They are collectively referred to as the jugular venous pulse and can provide clinically useful information about the heart. Atrial contraction produces the first pressure peak called the a wave. The c wave, which follows shortly thereafter, coincides with the onset of ventricular systole and is caused by an initial bulging of the tricuspid valve into the right atrium. Right atrial pressure falls after the c wave because of atrial relaxation and a downward displacement of the tricuspid valve during ventricular emptying. Right atrial pressure then begins to increase toward a third peak, the v wave, as the central veins and right atrium fill behind a closed tricuspid valve with blood returning to the heart from the peripheral organs. With the opening of the tricuspid valve at the conclusion of ventricular systole, right atrial pressure again falls as blood moves into the relaxed right ventricle. Shortly afterward, right atrial pressure begins to rise once more toward the next a wave, as returning blood fills the central veins, the right atrium, and the right ventricle together during diastole.
Heart Sounds
A phonocardiographic record of the heart sounds, which occur in the cardiac cycle, is included in Figure 3–1. These sounds are normally heard by auscultation with a stethoscope placed on the chest. The first heart sound, S1, occurs at the beginning of systole because of the abrupt closure of the AV valves, which produces vibrations of the cardiac structures and the blood in the ventricular chambers. S1 can be heard most clearly by placing the stethoscope over the apex of the heart. Note that this sound occurs immediately after the QRS complex of the electrocardiogram.
The second heart sound, S2, arises from the closure of the aortic and pulmonic valves at the beginning of the period of isovolumetric relaxation. This sound is heard near the end of the T wave in the electrocardiogram. The pulmonic valve usually closes slightly after the aortic valve. Because this discrepancy is enhanced during the inspiratory phase of the respiratory cycle, inspiration causes what is referred to as the physiological splitting of the second heart sound. The discrepancy in valve closure during inspiration may range from 30 to 60 ms. There are at least two factors that lead to this prolonged ejection time from the right ventricle during inspiration. The first is related to an inspiration-induced decrease in intrathoracic pressure and increased filling of the right side of the heart. This extra volume will be ejected, but a little extra time is required to do so. The second factor is related to the inspiration-induced decrease in pulmonary vascular resistance, which transiently reduces pulmonary artery pressure and right ventricular afterload. With reduced afterload, ventricular ejection can go on for a slightly longer period of time.
The third and fourth heart sounds, shown in Figure 3–1, are not normally present. When they are present, however, they, along with S1 and S2, produce what are called gallop rhythms (resembling the sound of a galloping horse). When present, the third heart sound occurs shortly after S2 during the period of rapid passive ventricular filling and, in combination with heart sounds S1 and S2, produces what is called ventricular gallop rhythm. Although S3 may sometimes be detected in normal children, it is heard more commonly in patients with left ventricular failure. The fourth heart sound, which occasionally is heard shortly before S1, is associated with atrial contraction and rapid active filling of the ventricle. Thus, the combination of S1, S2, and S4 sounds produces what is called an atrial gallop rhythm. The presence of S4 often indicates an increased ventricular diastolic stiffness, which can occur with several cardiac disease states.
There may be other sounds associated with the cardiac cycle that usually indicate abnormal conditions. Murmurs can occur during systole or diastole and usually indicate turbulent flow through cardiac valves that do not fully open or are completely closed. (These are described in more detail in Chapter 5.) Information about other abnormal sounds including rubs, snaps, and clicks can be obtained in more specific clinical references.
Cardiac Cycle Pressure–Volume and Length–Tension Relationships
Intraventricular pressure and volume are intimately linked to the tension and length of the cardiac muscle cells in the ventricular wall through purely geometric and physical laws. Figure 3–3A and 3–3B shows the correspondence between a ventricular pressure–volume loop and a cardiac muscle length–tension loop during a single cardiac cycle. These two loops indicate that cardiac muscle length–tension behavior is the underlying basis for ventricular function. Note that in Figure 3–3, each major phase of the ventricular cardiac cycle has a corresponding phase of cardiac muscle length and tension change. During diastolic ventricular filling, for example, the progressive increase in ventricular pressure causes a corresponding increase in muscle tension, which passively stretches the resting cardiac muscle to greater lengths along its resting length–tension curve. End-diastolic ventricular pressure is referred to as ventricular preload because it sets the end-diastolic ventricular volume and therefore the resting length of the cardiac muscle fibers at the end of diastole.
Figure 3–3. (A) Left ventricular pressure–volume cycle and (B) corresponding cardiac muscle length–tension cycle.
At the onset of systole, the ventricular muscle cells develop tension isometrically and intraventricular pressure rises accordingly. After the intraventricular pressure rises sufficiently to open the outlet valve, ventricular ejection begins as a consequence of ventricular muscle shortening. Systemic arterial pressure is often referred to as the ventricular afterload because it determines the tension that must be developed by cardiac muscle fibers before they can shorten.2
During cardiac ejection, the cardiac muscle is simultaneously generating active tension and shortening (ie, an afterloaded isotonic contraction). The magnitude of ventricular volume change during ejection (ie, stroke volume) is determined simply by how far ventricular muscle cells are able to shorten during contraction. This, as already discussed, depends on the length–tension relationship of the cardiac muscle cells and the load against which they are shortening. Once shortening ceases and the output valve closes, the cardiac muscle cells relax isometrically. Ventricular wall tension and intraventricular pressure fall in unison during isovolumetric relaxation.
DETERMINANTS OF CARDIAC OUTPUT
Cardiac output (liters of blood pumped by each of the ventricles per minute) is an extremely important cardiovascular variable that is continuously adjusted so that the cardiovascular system operates to meet the body’s moment-to-moment transport needs. In going from rest to strenuous exercise, for example, the cardiac output of an average person will increase from approximately 5.5 to perhaps 15 L/min. The extra cardiac output provides the exercising skeletal muscles with the additional nutritional supply needed to sustain an increased metabolic rate. To understand the cardiovascular system’s response not only to exercise but also to all other physiological or pathological demands placed on it, one must understand what determines and therefore controls cardiac output.
As stated in Chapter 1, cardiac output is the product of the heart rate and stroke volume (CO = HR × SV). Therefore, all changes in cardiac output must be produced by changes in the heart rate and/or stroke volume.
Factors influencing the heart rate do so by altering the characteristics of the diastolic depolarization of the pacemaker cells, as discussed in Chapter 2 (Figure 2–6). Recall that variations in activity of the sympathetic and parasympathetic nerves leading to cells of the sinoatrial (SA) node constitute the most important regulators of the heart rate. An increase in sympathetic activity increases the heart rate, whereas an increase in parasympathetic activity decreases the heart rate. These neural inputs have immediate effects (within 1 beat) and therefore can cause very rapid adjustments in cardiac output.
INFLUENCES ON STROKE VOLUME
Effect of Changes in Ventricular Preload: Frank–Starling’s Law of the Heart
The volume of blood that the heart ejects with each beat can vary significantly. One of the most important factors responsible for these variations in stroke volume is the extent of cardiac filling during diastole. This concept was introduced in Chapter 1 (Figure 1–7) and is known as Starling’s law of the heart. To review (and to reemphasize its importance), this law states that, with other factors equal, stroke volume increases as cardiac filling increases. As discussed in the following text, this phenomenon is based on the intrinsic mechanical properties of myocardial muscle.
Figure 3–4A illustrates how increasing muscle preload will increase the extent of shortening during a subsequent contraction with a fixed total load. Recall from the nature of the resting length–tension relationship that an increased preload is necessarily accompanied by increased initial muscle fiber length. As described in Chapter 2, when a muscle starts from a greater length, it has more distance to shorten before it reaches the length at which its tension-generating capability is no longer greater than the load on it. The same behavior is exhibited by cardiac muscle cells when they are actually operating in the ventricular wall. Increased ventricular preload increases both end-diastolic volume and stroke volume almost equally, as illustrated in Figure 3–4B.
Figure 3–4. The effect of an increase in preload on (A) cardiac muscle shortening during afterloaded contractions and (B) ventricular stroke volume.
The precise relationship between cardiac preload (cardiac filling pressure) and end-diastolic volume has especially important physiological and clinical consequences. Although the actual relationship is somewhat curvilinear, especially at very high filling pressures, it is nearly linear over the normal operating range of the heart. The low slope of this relationship indicates the substantial compliance of the normal ventricle during diastole (eg, a change in filling pressure of only 1 mm Hg normally will change end-diastolic volume by approximately 25 mL). As will be discussed in Chapter 11, one major form of cardiac failure is called “diastolic failure” and is characterized by a low ventricular compliance and a decidedly abnormal relationship between cardiac filling pressure and end-diastolic volume.
It should be noted in Figure 3–4A that increasing preload increases initial muscle length without significantly changing the final length to which the muscle shortens against a constant total load. Thus, increasing ventricular filling pressure increases stroke volume, primarily by increasing end-diastolic volume. As shown in Figure 3–4B, this is not accompanied by a significant alteration in end-systolic volume.
Effect of Changes in Ventricular Afterload
As stated previously, systemic arterial pressure is usually taken to be the left ventricular “afterload.” A slight complication is that arterial pressure varies between a diastolic value and a systolic value during each cardiac ejection. Usually, however, we are interested in mean ventricular afterload and take this to be mean arterial pressure.
Figure 3–5A shows how increased afterload, at constant preload, has a negative effect on cardiac muscle cell shortening. Again, this is simply a consequence of the fact that muscle cannot shorten beyond the length at which its peak isometric tension-generating potential equals the total load on it. Thus, shortening must stop at a greater muscle length when afterload is increased.
Figure 3–5. The effect of an increase in afterload on (A) cardiac muscle shortening during afterloaded contractions and (B) ventricular stroke volume.
Normally, mean ventricular afterload is quite constant because mean arterial pressure is held within tight limits by the cardiovascular control mechanisms described later. In many pathological situations such as hypertension and aortic valve obstruction, however, ventricular function is adversely influenced by abnormally high ventricular afterload. When this occurs, stroke volume may be decreased, as shown by the changes in the pressure–volume loop in Figure 3–5B. Under these conditions, note that stroke volume is decreased because end-systolic volume is increased.
The relationship between end-systolic pressure and end-systolic volume obtained at a constant preload but different afterloads is indicated by the dotted line in Figure 3–5B. In a normally functioning heart, the effect of changes in afterload on end-systolic volume (and therefore stroke volume) is quite small (approximately 0.5 mL/mm Hg). However, in what is termed “systolic cardiac failure,” the effect of afterload on end-systolic volume is greatly enhanced. Thus, the slope of this line can be used clinically to assess the systolic function of the heart, as discussed further in Chapter 11.
Effect of Changes in Cardiac Muscle Contractility
Recall that activation of the sympathetic nervous system results in release of norepinephrine from cardiac sympathetic nerves, which increases contractility of the individual cardiac muscle cells. This results in an upward shift of the peak isometric length–tension curve. As shown in Figure 3–6A, such a shift will result in an increase in the shortening of a muscle contracting with constant preload and total load. Thus, as shown in Figure 3–6B, the norepinephrine released by sympathetic nerve stimulation will increase ventricular stroke volume by decreasing the end-systolic volume, without directly influencing the end-diastolic volume.
Figure 3–6. The effect of an increase in contractility by norepinephrine (NE) on (A) cardiac muscle shortening during afterloaded contractions and (B) ventricular stroke volume.
The term ejection fraction is a clinically useful variable used to assess cardiac muscle contractility. It is the fraction of the blood in the ventricle at the end of diastole that is ejected during systole. It is defined as the ratio of stroke volume (SV) to end-diastolic volume (EDV):
As can be ascertained in Figure 3–6, increase and decrease in cardiac contractility cause increase and decrease in ejection fraction, respectively.3
In addition to this change in the extent of myocyte shortening, an increase in contractility will also cause an increase in the rates of myocyte tension development and of shortening. This will result in an increase in the rate of isovolumetric pressure development and the rate of ejection during systole.
SUMMARY OF DETERMINANTS OF CARDIAC OUTPUT
The major influences on cardiac output are summarized in Figure 3–7. The heart rate is controlled by chronotropic influences on the spontaneous electrical activity of SA nodal cells. Cardiac parasympathetic nerves have a negative chronotropic effect, and sympathetic nerves have a positive chronotropic effect on the SA node. Stroke volume is controlled by influences on the contractile performance of the ventricular cardiac muscle—in particular, its degree of shortening in the afterloaded situation. The three distinct influences on stroke volume are contractility, preload, and afterload. Increased cardiac sympathetic nerve activity tends to increase stroke volume by increasing the contractility of the cardiac muscle. Increased arterial pressure tends to decrease stroke volume by increasing the afterload on cardiac muscle fibers. Increased ventricular filling pressure increases end-diastolic volume, which tends to increase stroke volume through Starling’s law.
Figure 3–7. Summary of influences on cardiac output.
It is important to recognize at this point that both the heart rate and stroke volume are subject to more than one influence. Thus, the fact that increased contractility tends to increase stroke volume should not be taken to mean that, in the intact cardiovascular system, stroke volume is always high when contractility is high. Following blood loss caused by hemorrhage, for example, stroke volume may be low in spite of a high level of sympathetic nerve activity and increased contractility. The only other possible causes for low stroke volume are high arterial pressure and low cardiac filling pressure. Because arterial pressure is normal or low following hemorrhage, the low stroke volume associated with severe blood loss must be (and is) the result of low cardiac filling pressure.
Cardiac Function Curves
One very useful way to summarize the influences on cardiac function and the interactions between them is by cardiac function curves such as those shown in Figure 3–8.
In this case, cardiac output is treated as the dependent variable and is plotted on the vertical axis in Figure 3–8, while cardiac filling pressure is plotted on the horizontal axis.4
Figure 3–8. Influence of cardiac sympathetic nerve activity on cardiac function curves.
Different curves are used to show the influence of alterations in cardiac sympathetic nerve activity. Thus, Figure 3–8 shows how the cardiac filling pressure and the activity level of cardiac sympathetic nerves interact to determine cardiac output. When the cardiac filling pressure is 2 mm Hg and the activity of cardiac sympathetic nerves is normal, the heart will operate at point A and will have a cardiac output of 5 L/min. Each single curve in Figure 3–8 shows how cardiac output would be changed by changes in cardiac filling pressure if cardiac sympathetic nerve activity were held at a fixed level. For example, if cardiac sympathetic nerve activity remained normal, increasing cardiac filling pressure from 2 to 4 mm Hg would cause the heart to shift its operation from point A to point B on the cardiac function diagram. In this case, cardiac output would increase from 5 to 7 L/min solely as a result of the increased filling pressure (Starling’s law). If, on the other hand, cardiac filling pressure were fixed at 2 mm Hg while the activity of cardiac sympathetic nerves was moderately increased from normal, the heart would change from operating at point A to operating at point C. Cardiac output would again increase from 5 to 7 L/min. In this instance, however, cardiac output does not increase through the length-dependent mechanism because cardiac filling pressure did not change. Cardiac output increases at constant filling pressure with an increase in cardiac sympathetic activity for two reasons. First and most importantly, increased cardiac sympathetic nerve activity increases the heart rate. Second, increased sympathetic nerve activity increases stroke volume by increasing cardiac contractility.5
Cardiac function graphs thus consolidate knowledge of many mechanisms of cardiac control and are most helpful in describing how the heart interacts with other elements in the cardiovascular system. Furthermore, these graphs reemphasize the important point that a change in cardiac filling pressure alone will have a very potent effect on cardiac output at any level of sympathetic activity.
Summary of Sympathetic Neural Influences on Cardiac Function
Because of its importance in overall control of cardiac function, it is appropriate at this point to summarize the major direct effects that the sympathetic nervous system exerts on electrical and mechanical properties of the cardiac muscle and thus on cardiac pumping ability. These effects are initiated by norepinephrine interaction with β1-adrenergic receptors on cardiac muscle cells, resulting in a cascade of events involving the Gs activation of adenylate cyclase, formation of cAMP, and activation of protein kinase A with subsequent phosphorylation of many molecules that play key regulatory roles in intracellular processes. These cellular events resulting from increase in sympathetic neural activity to the heart combine to evoke improvements in pumping capabilities of the heart. These improvements include the following:
1. an increase in the heart rate (positive chronotropic effect) by activating the inward-going if current in SA nodal cells;
2. a decrease in cardiac action potential duration by early activation of the delayed iK current in cardiac myocytes, which minimizes the detrimental effect of high heart rates on diastolic filling time;
3. an increase in the rate of action potential conduction, particularly evident in the AV node (positive dromotropic effect) by altering conductivity of gap junctions and by increasing the rate of initial depolarization of the action potential;
4. an increase in cardiac contractility (positive inotropic effect) by activating the iCa2+ current and increasing Ca2+ release from the sarcoplasmic reticulum, which increases the contractile ability of the cardiac muscle at any given preload; and
5. an increase in the rate of cardiac relaxation (positive lusitropic effect) by increasing Ca2+ uptake by the sarcoplasmic reticulum, which also helps minimize the detrimental effect of high heart rates on diastolic filling time.6,7
As will be presented in subsequent chapters, increases in sympathetic activity can have indirect influences on cardiac function that are a consequence of sympathetic-induced alterations in arteriolar and venous tone (ie, alterations in afterload and preload, respectively).
CARDIAC ENERGETICS
Energy Sources
For the heart to operate properly, it must have an adequate supply of chemical energy in the form of adenosine triphosphate (ATP). The relatively low ATP content of cardiac tissue combined with a relatively high rate of ATP hydrolysis at rest suggests that the myocardial ATP pool will completely turn over every 10 s.
The substrates from which ATP is formed by the heart depend partly on which substrates are in the greatest supply at a particular instant. For example, after a high-carbohydrate meal, the heart will take up and metabolize glucose and pyruvate, whereas between meals, the heart can switch to metabolize free fatty acids, triglycerides, and ketones. Unlike the skeletal muscle, the cardiac muscle can utilize lactate as an energy source, which is beneficial during strenuous exercise when skeletal muscles are producing lactate. In addition, the choice of substrate depends on the metabolic phenotype of the cardiac muscle. Fetal and newborn hearts derive most of their ATP from metabolism of glucose and lactate, whereas within a few weeks of birth a switch toward fatty acid oxidation occurs so that by adulthood 60% to 90% of cardiac ATP is derived from fatty acids. A switch back toward the fetal phenotype accompanies severe heart failure. Glycogen is stored in myocardial cells as a reserve energy supply and can be mobilized via the glycolytic pathway to provide extra substrate under conditions of increased sympathetic stimulation.
The end product of metabolism of glycogen, glucose, fatty acids, triglycerides, pyruvate, and lactate is acetyl CoA, which enters the citric acid (Krebs) cycle in the mitochondria, where, by a process of oxidative phosphorylation, the molecules are degraded to carbon dioxide and water and the energy is converted to ATP. (The student is encouraged to consult a biochemistry textbook for further details of these important metabolic pathways.)
The anaerobic sources of energy in the heart (eg, glycolysis and creatine phosphate) are not adequate to sustain the metabolic demand for more than a few minutes. The heavy (nearly total) reliance of the heart on the aerobic pathways for ATP production is evident by (1) the high number of mitochondria and (2) the presence of high concentrations of the oxygen-binding protein myoglobin within the cardiac muscle cells. Myoglobin can release its oxygen to the mitochondrial cytochrome oxidase system when intracellular oxygen levels are lowered. In this regard, the cardiac muscle resembles “red” skeletal muscle that is adapted for sustained contractile activity as opposed to “white” skeletal muscle that is adapted for high-intensity, short-duration contractile activity.
Determinants of Myocardial Oxygen Consumption
In many pathological situations, such as obstructive coronary artery disease, the oxygen requirements of the myocardial tissue may exceed the capacity of coronary blood flow to deliver oxygen to the heart muscle. It is important to understand what factors determine the energy costs and, therefore, the myocardial oxygen consumption rate because reduction of the oxygen demand may be of significant clinical benefit to the patient.
Because the heart derives its energy almost entirely from aerobic metabolism, myocardial oxygen consumption is directly related to myocardial energy use (ie, ATP splitting). Understanding the determinants of myocardial oxygen consumption essentially means understanding the myocardial processes that require ATP.
The basal metabolism of the heart tissue normally accounts for approximately 25% of myocardial ATP use and therefore myocardial oxygen consumption in a resting individual. Because basal metabolism represents the energy consumed in cellular processes other than contraction (eg, energy-dependent ion pumping), little can be done to reduce it.
The processes associated with muscle contraction account for approximately 75% of myocardial energy use. Primarily, this reflects ATP splitting associated with cross-bridge cycling during the isovolumetric contraction and ejection phases of the cardiac cycle. Some ATP is also used for Ca2+ sequestration at the termination of each contraction.
The energy expended during the isovolumetric contraction phase of the cardiac cycle accounts for the largest portion (~50%) of total myocardial oxygen consumption despite the fact that the heart does no external work during this period. The energy needed for isovolumetric contraction depends heavily on the intraventricular pressure that must develop during this time, that is, on the cardiac afterload. Cardiac afterload then is a major determinant of myocardial oxygen consumption. Reductions in cardiac afterload can produce clinically significant reductions in myocardial energy requirements and therefore myocardial oxygen consumption.
Energy utilization during isovolumetric contraction is actually more directly related to isometric wall tension development than to intraventricular pressure development. Recall that wall tension is related to intraventricular pressure and ventricular radius through the law of Laplace (T = P × r). Consequently, reductions in cardiac preload (ie, end-diastolic volume and radius) will also tend to reduce the energy required for isovolumetric contraction.
It is during the ejection phase of the cardiac cycle when the heart actually performs external work, and the energy the heart expends during ejection depends on how much external work it is doing. In a fluid system, work (force × distance) is equal to pressure (force/distance2) × volume (distance3). The external physical work done by the left ventricle in 1 beat, called stroke work, is equal to the area enclosed by the left ventricular pressure–volume loop (see Figure 3–3). Stroke work is increased either by an increase in stroke volume (increased “volume” work) or by an increase in afterload (increased “pressure” work). In terms of ATP utilization and oxygen consumption, increases in the pressure work of the heart are more costly than increases in volume work. Thus, reductions in afterload are especially helpful in reducing the myocardial oxygen requirements for doing external work.
Changes in myocardial contractility can have important consequences on the oxygen requirement for basal metabolism, isovolumic wall tension generation, and external work. Heart muscle cells use more energy in rapidly developing a given tension and shortening by a given amount than in doing the same thing more slowly. Also, with increased contractility, more energy is expended in active Ca2+ transport. The net result of these influences is often referred to as the “energy wasting” effect of increased contractility.
The heart rate is one of the most important determinants of myocardial oxygen consumption because the energy cost per minute must equal the energy cost per beat times the number of beats per minute. In general, it has been found that it is more efficient (ie, less oxygen is required) to achieve a given cardiac output with the low heart rate and high stroke volume than with the high heart rate and low stroke volume. This again appears to be related to the relatively high energy cost of the pressure development phase of the cardiac cycle.
PERSPECTIVES
The job of the heart is to establish the pressure that drives blood passively through the pulmonic and systemic circulations. This is a remarkable, highly efficient, adaptable, and long-lasting pump that we, despite our best efforts, are unable to duplicate with any significant degree of success. When it breaks down, we suffer rather immediate adverse consequences.
As might be expected, support of this pump is highly dependent upon maintenance of coronary flow to the ventricular wall. Much of our current medical interventions are aimed at the coronary vasculature. Description of coronary flow is presented in more detail in Chapter 8.
In this book, we have ignored the extracellular structures of the heart, that is, the fibrous valves, the connective tissue frame (cardiac skeleton) that functions to electrically isolate the atria from the ventricles, and the extracellular matrix that forms a dynamic scaffolding surrounding the contractile cells. These structures are made primarily of collagen from the fibroblasts and not only maintain the structural integrity of the heart but appear to participate importantly in dynamic adaptations to changing conditions. There is current interest in the influence of the extracellular matrix components on cardiac behavior.
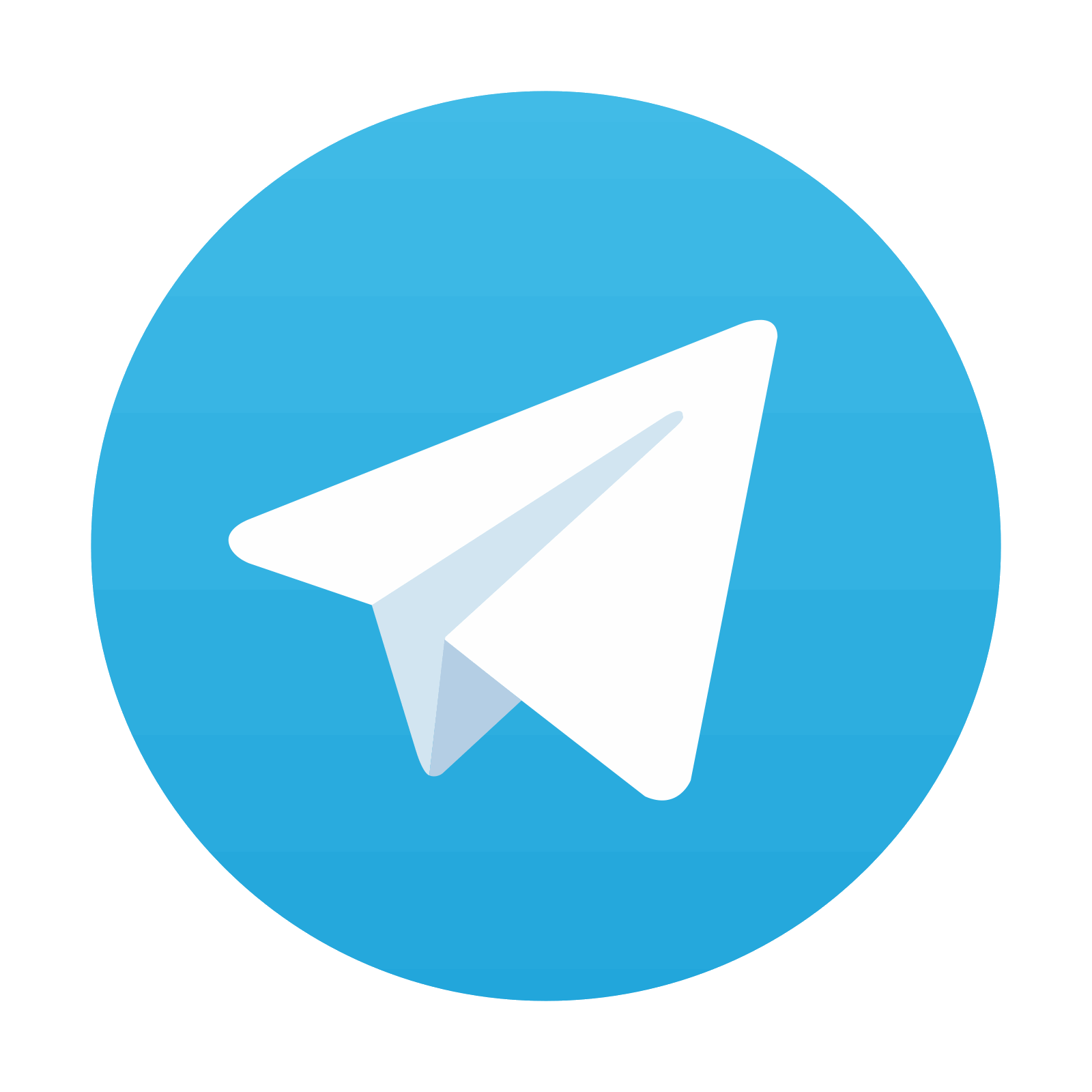
Stay updated, free articles. Join our Telegram channel
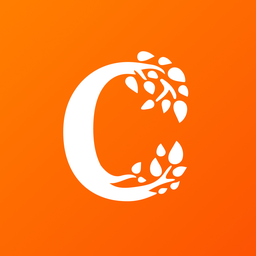
Full access? Get Clinical Tree
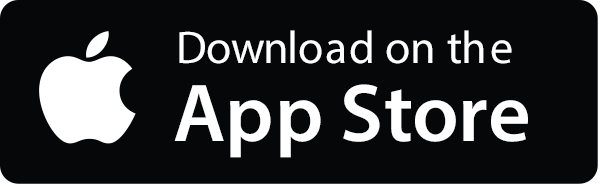
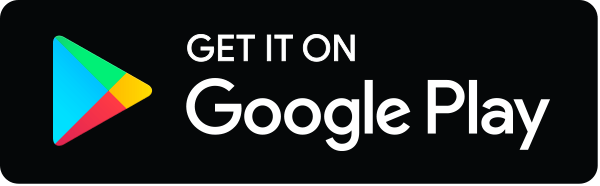