CHAPTER 109 Surgical Approaches and Cardiopulmonary Bypass in Pediatric Cardiac Surgery
Thoracic incisions traditionally used in adult cardiac and thoracic surgery have been used in children with varying success with respect to exposure, pain, and cosmetic result. Special considerations relevant to pediatric surgery are related to the lack of development and growth of soft tissue structures such as breast tissue, and bony structures such as ribs and vertebra. Thus, incisions that fix growing bony structures, such as ribs in a posterior or lateral thoracotomy, may lead to scoliosis.1 Anterior thoracic incisions may also injure underdeveloped breast tissue and pectoral muscles, resulting in chest wall deformities and sensory loss. Then again, the flexibility of the chest cage and ribs in young children permit the use of limited incisions with adequate exposure of relevant structures, whereas in adults this may not be possible without the risk of rib or sternal fracture or instability of the chest cage. Therefore, these factors should be considered when selecting the optimal approach to intrathoracic structures in children, to optimize exposure and for the safe conduct of the procedure, and to minimize pain and achieve a cosmetically acceptable result.
APPROACHES TO CARDIAC STRUCTURES AND GREAT VESSELS FOR TRANSTHORACIC CANNULATION FOR BYPASS
Limited Sternotomy Incisions
For many procedures, a full sternotomy is not necessary to provide adequate access to all the relevant structures of the heart. This is particularly true in infants and young children because their sternum and rib cage are pliable, which permits retraction with minimal force. Preoperative planning of the procedure includes determining which mediastinal structures will need exposure for the surgical procedure and for cannulation for cardiopulmonary bypass (CPB). With the availability of thin-walled, wire-reinforced, small-diameter cannulas for use in children, cannulation for CPB can often be achieved with minimal extension of the thoracic incision beyond that needed for the repair itself. These approaches usually provide exposure sufficient to use standard techniques for myocardial protection, such as cardioplegia and left ventricular venting. A full sternotomy is unnecessary when, for example, the intracardiac repair is accomplished via a right atriotomy, such as for repair of atrial septal defect, ventricular septal defect, or complete atrioventricular canal defect, and in transatrial repair of tetralogy of Fallot and mitral valve repair.2–4
Trans-Xyphoid Mini-Sternotomy
With the trans-xyphoid mini-sternotomy approach, the skin incision extends from the level of the areola (mid thorax) down to the tip of the xyphoid process (Fig. 109–1). By detaching anterior diaphragm attachments to the cartilaginous segment of the rib cage anteriorly, access is gained to the anterior mediastinum. Before performing the partial sternotomy, blunt dissection is required to detach the pericardium and thymus from the sternum. A partial sternotomy can be performed with a saw, but in infants, heavy bandage scissors are sufficient for dividing the lower sternum. Once the partial sternotomy is completed, a narrow-blade retractor such as an army-navy retractor is used to lift the sternum anteriorly and cephalad to provide exposure to the ascending aorta for cannulation. The retractor should not be placed on the skin and subcutaneous tissue, as adequate exposure of the upper mediastinum will not be achieved and necrosis of the skin could occur from prolonged retraction. To expose the aorta, however, caudal and anterior traction must be placed on the pericardium. This maneuver requires that thymus attachments to the pericardium be divided, or mobility of the pericardium and aorta will be inadequate. The pericardial incision must be made to the right of midline, and the pericardium should be pinned to the right edge of the divided sternum to expose the right atrium and cavae. In most cases, cannulation of the ascending aorta and both cavae can be easily achieved with this approach. Insertion of the inferior caval cannula through a separate skin incision keeps the cannula out of the way, and the incision can later be used for tube thoracostomy (Fig. 109–2).
Anterior or Anterolateral Thoracotomy
An anterior thoracotomy has been advocated for surgical repair of atrial septal defect and occasionally ventricular septal defects.5,6 The incision is made in the anterior fourth intercostal space (ICS), and in females great care must be taken to be well below breast tissue. Dissection of the pericardial attachments to the sternum and thymus greatly facilitates exposure by permitting retraction of the pericardium down toward the diaphragm and bringing the ascending aorta closer into view. Direct cannulation of the ascending aorta is preferable to peripheral cannulation via the femoral or axillary artery, as these vessels are small in children and stenosis at the cannulation site can result in claudication with exercise. Recently, more flexible cannulas, available in all sizes for pediatric use, have facilitated aortic cannulation. Some arterial cannulas can be introduced over a guide wire, which makes insertion easier and safer. The cavae can be cannulated directly, although the superior cava cannula is often best introduced via the right atrial appendage and directed retrograde into the superior vena cava (SVC). Aortic clamping to achieve cardiac arrest can be difficult with conventional arterial clamps, which were designed for application via a sternotomy. In small children, a bulldog clamp is sufficient for aortic occlusion, and for larger children and teenagers, flexible clamps are now available. Cardioplegia can be delivered via a small flexible cannula inserted into the aortic root; some have advocated transthoracic insertion of a needle into the aortic root.
APPROACHES TO EXTRACARDIAC STRUCTURES IN INFANTS AND CHILDREN
Noncardiac thoracic surgery for the treatment of congenital cardiac defects or complications of cardiac surgery most often requires exposure to the posterior mediastinum. Exceptions include procedures to plicate the diaphragm, or for unifocalization of aortopulmonary collaterals, which requires dissection of the hilum of the lung. A posterolateral incision extending from just anterior to the tip of the scapula to the mid-posterior scapula provides access, through either the fourth, fifth, or sixth ICS, to posterior mediastinal structures as well as the hilum of the lung, the pericardium, and the diaphragm (Fig. 109–3). If the upper half of the mediastinum needs exposure, an incision through the fourth ICS is optimal. For access to the hilum of the lung, the fifth ICS is optimal, and, for access to the thoracic duct at the level of the diaphragm, or to the central tendon of the diaphragm, a sixth ICS incision is optimal. The most common procedures in which a thoracotomy is performed routinely are operations on the thoracic aorta or branches, such as surgery for repair of coarctation of the aorta or for ligation of the patent ductus. Care should be taken to ensure that the appropriate interspace is entered, as exposure to the upper thorax can be very difficult in small children if the fifth interspace, or lower, is entered. Although counting ribs starting at the second rib, which has attachments of the anterior scalene muscle, can be done, external landmarks can also be used. A useful landmark is the position of the areola when the arm is extended over the head. With the arm extended, the interspace below the areola is usually the fourth ICS, and this landmark can confirm a particular interspace identified by other techniques. This method for identification of interspace is particularly useful in neonates and premature infants. Extensive division of the intercostal muscle is usually unnecessary in infants and small children, as adequate exposure can be obtained by separating the ribs, which are more flexible in this age group and entail less risk for fracture.
Thoracoscopic Approach in Children
Because most cardiac procedures in children require CPB and intracardiac repair, port access for reconstruction has been applied almost exclusively to adult patients or, rarely, adult-size teenagers. Thoracoscopic procedures in children have been for the most part confined to approaches to noncardiac structures or to the pericardium. Examples include ligation of the patent ductus, division of vascular rings, creation of a pericardial window, and, more recently, insertion of pacer leads.7,8 Much of the instrumentation has been adapted from other surgical applications, and the thoracoscopic procedures have involved primarily dissection and ligation or division with little reconstruction or suturing. Using induced electromyography to establish the location and local course of the recurrent laryngeal nerve may be of some benefit in infants and small children undergoing patent ductus arteriosus ligation or vascular ring procedures.9
Positioning and location of port incisions follows the principles of thoracoscopy or thoracotomy in adults. For access to the distal transverse aortic arch and descending aorta, the patient should be in a full lateral decubitus position. For access to anterior mediastinal structures, such as the anterior pericardium or thymus, a partial decubitus position with the thorax tilted toward a supine position is optimal. Usually, four incisions are required, two for the surgeon’s instruments for the dissection, one for the scope and camera, and the fourth for the assistant to introduce lung retractors or occasionally a grasper or suction. As with any thoracoscopic procedure, the central port is used for the camera and the instrument ports are to each side of the camera port, separated by sufficient distance to prevent the scope from interfering with instrument movement. When a surgical robot is used to assist, the same port position is used but the port for the lung retractor and suction is placed at the midaxillary line at the sixth or seventh ICS (Fig. 109–4).
CARDIOPULMONARY BYPASS IN CHILDREN
History
The use of extracorporeal circulatory techniques in the repair of congenital cardiac defects began in the 1950s, shortly after the concept of CPB appeared and a heart–lung machine was constructed. Gibbon repaired an atrial septal defect using the first heart–lung machine, which required 12 to 14 units of blood prime, in 1953.10 At approximately the same time, Lillihei and colleagues began using cross-circulation to repair a variety of defects in relatively young infants and children, including ventricular septal defects, atrioventricular canal defects, and tetralogy of Fallot, achieving a remarkable overall survival of greater than 60%.11 Shortly thereafter, Kirklin developed a pump oxygenator derived from Gibbon’s earlier efforts.12 This device required approximately half of the original amount of fresh blood prime but also extreme care to prevent severe foaming of blood, which was lethal; nonetheless, the survival rate was 50%. These early reports prompted many subsequent investigations aimed at developing the scientific and technologic knowledge needed to successfully undertake extracorporeal circulation in infants and children. In spite of these efforts, the morbidity and mortality of CPB remained high throughout the 1960s.
The next major advance occurred in the early 1970s, when Barratt-Boyes and Castaneda described the use of deep hypothermic circulatory arrest in infants.13,14 These techniques relied primarily on surface cooling, with exposure to the CPB circuit limited to a brief period of core cooling and rewarming, so that total CPB time was typically kept under 20 to 30 minutes. Progressive advances in the design of circuit components and perfusion techniques for infants and small children occurred throughout the 1980s and into the early 1990s. As a result, the “toxicity” associated with the use of CPB in infants declined significantly, to the point where lengthy and complex repairs such as the arterial switch procedure for transposition of the great arteries and primary repair of tetralogy of Fallot can currently be undertaken in neonates and very young infants using CPB with an overall mortality rate of less than 5%. Nonetheless, the morbidity associated with the use of CPB in infants and children is still widely held to be a major limitation to completely successful outcomes.
Differences between Pediatric and Adult CPB
There are many significant differences in circuit technology and the physiologic effects of CPB in neonates, infants, and small children compared with adults. The surface area and volume of the CPB circuit relative to patient size and blood volume is much greater for neonates and infants. Arterial and venous cannulas are smaller but more likely to deform or obstruct the aorta or venae cavae; their placement can be different and more variable than in adults—for example, separate superior and inferior vena caval cannulas, or initial placement of the aortic cannula in the pulmonary artery (with retrograde systemic perfusion via the ductus arteriosus) during stage I repair of hypoplastic left heart syndrome. To minimize hemodilution, the sizes of various circuit components and tubing diameters are kept as small as possible. Nonetheless, hemodilution that is equivalent to one to two blood volumes from the circuit prime and cardioplegia is fairly common in neonates and small infants (see Table 109-1).
Patient Factors
Patient-specific variables and the diverse pathophysiology associated with specific congenital cardiac defects further complicate CPB in neonates, infants, and small children. It is likely that neonates, in general, and particularly those who are premature or weigh less than 1.8 to 2.0 kg, comprise a high-risk group because of immature organ function, as well as coexisting diseases such as sepsis, respiratory distress syndrome, and other congenital anomalies.15 The immature myocardium may be similarly prone to CPB-related dysfunction for several reasons, including its relatively deficient (compared with in adults) contractile protein mass and organization of contractile proteins, the presence of fetal contractile protein isoforms, immature calcium cycling (which occurs primarily via the sarcolemmal membrane as opposed to the sarcoplasmic reticulum, which is less abundant and less well organized), and fewer mitochondria.
Various aspects of congenital heart disease can mean additional complicating features. Hypertrophic and cyanotic myocardium is more likely to be injured by ischemia-reperfusion and other consequences of CPB.16–19 Aortopulmonary collaterals, which can be particularly significant in various cyanotic lesions, may promote pulmonary dysfunction as a result of high flow on CPB, whereas steal from systemic perfusion can compromise the function of other organs, and collaterals to the coronary circulation can wash out cardioplegia and thereby hinder effective myocardial preservation. Pulmonary dysfunction after CPB may be more prevalent in infants with other routes of high pulmonary blood flow (e.g., truncus arteriosus, hypoplastic left heart syndrome, transposition of the great arteries) and in cyanotic infants.15,20 Diffuse organ dysfunction is likely to be more common in patients who were severely cyanotic and hypoperfused at the time of delivery or who required complex surgery in the early neonatal period. In the neonate who was compromised at the time of delivery or thereafter, most centers have found it beneficial to allow a period of stabilization of the circulation and recovery of organ function prior to undertaking CPB and cardiac surgery, using lesion-appropriate interventions such as prostaglandin E1, inotropic support, ventilatory strategies to balance systemic and pulmonary blood flow, and even extracorporeal circulatory support (see later). Post-CPB organ dysfunction (e.g., kidney, liver) can also be a source of morbidity in older (i.e., adult-age) patients with various forms of congenital heart disease complicated by long-standing cyanosis, low cardiac output, or high systemic venous pressures.
Differences in the Cardiopulmonary Bypass Circuit
A summary of the components of different-sized CPB circuits is shown in Table 109-1.
Oxygenators
Virtually all current pediatric CPB applications use membrane-type oxygenators. The two main types are microporous (hollow-fiber or folded-membrane) and nonporous membrane oxygenators. The major advantage of microporous-type membranes is their ability to effect gas exchange with a relatively modest membrane surface area, typically in the range of 0.2 to 1.5 m2, depending on the specific oxygenator and configuration. Major disadvantages include some blood–gas contact at the start of CPB (until protein accumulation blocks the 0.05- to 0.25-μm pores) and protein leakage across the membrane, along with the potential for gas embolization if negative pressure develops on the blood side of the artificial membrane. Nonporous oxygenator membranes, typically of the folded-sheet silicone-membrane variety, require a larger surface area to achieve gas exchange but do not accumulate or leak protein as readily and are therefore more often selected for longer-term circulatory support applications (e.g., extracorporeal membrane oxygenation).
There is a real need to minimize circuit priming volume to minimize hemodilution, blood product exposure, and the potential for fluid overload and edema. Priming volume is usually defined as the volume of the membrane compartment plus the minimal amount required for the venous reservoir. Typical priming volumes of commercial membrane oxygenators range from approximately 225 to 375 mL when used in the open configuration. The Dideco Lilliput hollow-fiber membrane oxygenator (Dideco, Mirandola, Italy) is an example of one with a smaller prime volume (about 70 mL), but it is not available in an open-system configuration. Various schema for achieving low priming volume and thereby reducing or avoiding the need for blood products as a component of the priming solution have been described.21–24
Tubing
Competing considerations govern selection of tubing size for infant and pediatric CPB (Table 109-2). The internal diameter needs to be large enough to permit the required full flow rate (see later) without inordinately increasing circuit line pressures. On the other hand, the tubing should be as narrow and as short as practical, to minimize priming volume. Some neonatal circuits use 1⁄4-inch tubing on both the arterial and venous limbs, and most centers have further decreased the diameter of the arterial tubing to 3⁄16 inch; some use 3⁄16 inch for both arterial and venous limbs (although vacuum-assisted venous drainage may be required in this instance).15 The pump is usually situated as close to the surgical field as possible to reduce tubing length, which is a major contributor to overall circuit volume.
Table 109–2 Infant and Pediatric Cardiopulmonary Bypass Tubing
Tubing Diameter (inch) | Tubing Volume (mL/ft) | Flow Rate (mL/revolution) |
---|---|---|
![]() | 5.0 | 7.4 |
¼ | 9.7 | 13 |
⅜ | 21.7 | 27 |
½ | 38.6 | 45 |
Venous Drainage
Vacuum-assisted venous drainage has been used for pediatric patients.15,22,25 Although this has not been well studied in a controlled or prospective fashion, advantages may include the ability to reduce total circuit volume by using lower venous reservoir volume and 3⁄16-inch venous tubing, improving operating conditions to some extent by allowing use of smaller venous cannulas, and improving venous drainage through the small cannulas and tubing. Theoretically, the improvement of venous drainage could lead to reduced tissue and organ edema and congestion, improved organ function, and reduced inflammatory activation (e.g., via endotoxin release from congested, hypoperfused intestine). A major potential complication of vacuum-assisted venous drainage is venoarterial air embolism.26–30
Arterial Cannulas
Issues regarding cannula size are much more significant in infants and small children than in adults. Arterial cannulas for neonates and small infants must be of sufficient size to permit appropriate arterial inflow rates at reasonable line pressures, with minimal shearing or jetting of blood flow (which can damage the vessel intima, aortic valve, or blood elements), yet small enough to fit in small aortas without obstructing aortic flow (Table 109-3). Small cannulas (i.e., 8 French) with a thin-walled, reinforced design that prevents cannula kinking and allows a larger luminal diameter for a given external diameter have become popular choices for infant bypass (Biomedicus). Care also needs to be taken not to kink or compress the infant’s pliable aorta by the location and orientation of the aortic cannula and its tubing. As noted, the cannula itself can significantly obstruct flow in the vessel around the cannula. Location of the tip can direct blood flow toward or away from specific vessels; for example, locating and directing the tip more distally toward the transverse arch may reduce flow through the right carotid artery, or favor lower body perfusion at the expense of cerebral perfusion and optimal brain cooling.31
Table 109–3 Representative Pediatric Arterial Cannulas
Weight (kg) | Standard Arterial Size (French) | Biomedicus Pediatric Arterial Size (French) |
---|---|---|
<5 | 10 | 8 |
5-10 | 12 | 10 |
10-14 | 14 | 12 |
14-28 | 16 | 14 |
28-40 | 18 | 15 |
40-55 | 20 | 17 |
Venous Cannulas
Choice and location of venous cannulas can also be more complex and variable than is typically the case in adults, and variations in venous anatomy and drainage as well as the operative approach must be taken into account. Venous cannulas are available in a variety of sizes and with design features for particular indications (Table 109-4). For example, thin-walled cannulas with multiple side holes enhance venous drainage; right-angled tips are frequently used in the SVC to improve alignment (and therefore drainage) and minimize impact on the surgical field; metal-tipped, to prevent kinking; straight, short-tipped in the IVC, to limit hepatic venous obstruction.
Table 109–4 Representative Venous Cannulas for Pediatric Cardiopulmonary Bypass
Weight (kg) | Single Venous (French) |
---|---|
<3.5 | 14 |
3.5-5 | 14 |
5-8 | 16 |
8-12 | 16 |
12-18 | 18 |
18-26 | 20 |
26-55 | 22 |
>55 | 24 |
Weight (kg) | Metal-Tip, Right-Angle Venous∗ (French) | |
---|---|---|
SVC | IVC | |
<3 | 12 | 12 |
3-6 | 12 | 14 |
6-8 | 12 | 16 |
8-12 | 14 | 16 |
12-16 | 14 | 18 |
16-22 | 16 | 18 |
22-30 | 16 | 20 |
30-34 | 18 | 20 |
34-46 | 18 | 20-22 |
46-58 | 20 | 22 |
Weight (kg) | Dual-Stage† (French) |
---|---|
<12 | 18/24 |
12-30 | 20/28 |
30-65 | 29/29 |
>65 | 29/37∗ |
Weight (kg) | Dual-Stage with Venous Assist (French) |
---|---|
<20 | 18/24 |
20-45 | 20/28 |
45-85 | 29/29 |
>85 | 29/37 |
IVC, inferior vena cava; SVC, superior vena cava.
∗ For example, the Medtronic DLP (Minneapolis, MN).
† For example, Edwards Lifesciences (Irvine, CA).
Regardless of location, it is important that the cannula be appropriately sited and that it cause as little obstruction to venous drainage as possible. Poor venous drainage may be difficult to detect and is more likely to occur in patients with complicated venous anatomy. The consequences of impaired venous drainage and increased venous pressures are likely to be magnified during CPB because arterial perfusion pressure is frequently reduced. Obstruction of the SVC may promote cerebral edema and otherwise increase the risk of brain injury by decreasing cerebral blood flow (CBF) and hindering effective brain cooling. Many practitioners find monitoring of SVC pressure by a catheter placed in the internal jugular vein useful to detect possible SVC obstruction in this setting; the patient’s head and face should also be inspected at regular intervals during CPB for the appearance congestion or swelling. It is likely that monitoring CBF velocity using transcranial Doppler methodology might also be useful in this regard. IVC obstruction will increase lower body venous pressures and potentially decrease hepatic, renal, or mesenteric perfusion; isolated hepatic vein obstruction can also occur. Consequences can include hepatic dysfunction, renal dysfunction, ascites, and perhaps increased inflammatory consequences of mesenteric congestion.32,33 IVC obstruction during CPB can be difficult to detect and should be suspected whenever there is development of ascites, decreased urine output, or impaired venous return.
Filters
Almost all applications use 0.2-μm filters in the gas inflow lines to prevent bacterial or particulate contamination. Similarly, all crystalloid prime and cardioplegia solutions are passed through 0.2-μm filters prior to final addition to the CPB circuit. A 20- or 40-μm filter is used on the cardiotomy suction return line to remove macroaggregates and microaggregates and other debris from the blood returning from the surgical field. Exogenous blood is also filtered (typically through a 40-μm blood filter) before it is added to either the pump circuit or to cardioplegia. Specific removal of blood polymorphonuclear leukocytes using leukocyte-depleting filters placed in-line on the CPB circuit (which typically reduces circulating leukocyte counts in the patient by about 75%) is advocated by some centers as a significant means to reduce reperfusion injury.20,34
Use of an arterial filter (40 μm) is somewhat more controversial. Many consider arterial filtering essential to limit the microemboli and amount of other debris from platelet and leukocyte microaggregates, traumatized blood elements, fat, and other sources, particularly as these might contribute to post-CPB neurologic injury and damage to other organ systems. Some practitioners do not feel so strongly about these issues and omit arterial line filters, at least in part to reduce priming volume and hemodilution.15
CPB Prime
The use of albumin or other colloid to prime CPB circuits is also controversial.35–37 There is evidence that reduced plasma protein concentrations and diminished plasma oncotic pressure can reduce lymphatic flow and increase capillary leak in the lung and other vascular beds.38–41 Although fluid balance and weight gain were favorably influenced, a recent study that randomized pediatric CPB patients to crystalloid or colloid prime could not demonstrate significant differences in mortality or in length of mechanical ventilation, intensive care unit stay, or hospital stay.41 These results are similar to those obtained in adults, where albumin does prevent CPB-induced reductions in colloid oncotic pressure and lung water accumulation, but it appears to have little affect on overall outcome or measures of pulmonary, myocardial, or renal function.
Hemodilution
Both packed red cells and whole blood have been used to ensure age-, lesion-, and temperature-appropriate hematocrit during CPB. Packed red cells are readily available and are probably used by most centers to increase and maintain hematocrit during CPB. A major disadvantage of whole blood compared with packed red cells is the higher glucose load that accompanies whole blood; hyperglycemia may increase the risk of brain injury during cerebral ischemia. On the other hand, whole blood more effectively maintains plasma factor concentrations, which can be significantly reduced by CPB in these patients (see later). Questions about the actual oxygen carrying and delivery capacity of stored red blood cells have been raised.42
Despite these theoretical considerations, the optimal and maximal tolerable levels of hemodilution for a given degree of hypothermia are not established. Normovolemic hemodilution with hematocrit levels down to 15% are believed to be well tolerated during normothermia in terms of cerebral and myocardial function as long as blood pressure, oxygenation, and cardiac output are maintained.43,44 Long-standing practice at many centers has been to aim for hematocrits in the 18% to 22% range during deep hypothermic CPB, and this is based on the aforementioned improvements in blood rheology and overall oxygen-carrying capacity that accompany the combination of hemodilution and hypothermia. Animal studies and reports of children of the Jehovah’s Witness faith undergoing hypothermic CPB suggest no detectable effect on overall outcome or cerebral or cardiovascular morbidity at hematocrits in the range of 10% to 18%, as long a low temperature, perfusion pressure, and flow rate are maintained.44–47 Hematocrits of 10% or less in infants were associated with acidosis and other evidence of inadequate oxygen delivery.
More recent evidence has cast doubt on the safety of very low hematocrits during hypothermic CPB in infant patients. In experimental infant CPB models, higher hematocrit (in the 25% to 30% range) has been associated with enhanced preservation of brain high-energy phosphates, intracellular pH, tissue oxygenation, maintained capillary density and microvascular flow, reduced leukocyte activation, and reduced neurologic injury.48–50 A recent clinical study that randomized infants to either a low hematocrit (mean hematocrit, 22% ± 3%) or high hematocrit (28% ± 3%) strategy at the start of low-flow hypothermic CPB found lower postoperative cardiac index, higher serum lactate, and higher total body water in the low hematocrit group. At 1 year, overall neurologic evaluations and mental development index scores were similar in the two groups, but the low hematocrit group had significantly lower psychomotor development index scores.51 Our current practice is to aim to keep the hematocrit between approximately 25% and 30% during all types of CPB. A hematocrit level at the onset of low-flow CPB of around 25% was associated with higher psychomotor development index scores and reduced lactate levels.52,53 In addition to any direct effects, it is likely that the somewhat higher hematocrit provides some degree of safety margin against other problems with perfusion, collaterals, and alterations in cerebral autoregulation and CBF.46,52–57 However, similar variations in patient age, anatomy, collaterals, flow rate, pH strategy, and cooling, for example, make it impossible to pronounce a single optimal hematocrit for infants or children.
Pump Flow Rates during Pediatric CPB
Optimal pump flow rates for pediatric CPB are, as for adults, based on considerations of adequate systemic oxygenation, oxygen delivery, and organ perfusion at normothermia as assessed by oxygen consumption and metabolic rate, mixed venous oxygen saturation, acid–base balance, and lactate production.58 These are typically indexed to body weight. The higher metabolic rate of neonates and infants (approximately 1.5- to 2.5-fold greater than adults) mandates proportionately higher flow rates during normothermic CPB (Table 109-5).
Table 109–5 Estimated Flow Rates for Normothermic Infant and Pediatric Cardiopulmonary Bypass
Body Weight (kg) | Flow Range (mL/kg/min) | Usual Full-Flow Rate (mL/kg/min) |
---|---|---|
<3 | 150-200 | 200 |
3-10 | 125-175 | 150 |
10-15 | 120-150 | 125 |
13-30 | 80-120 | 100 |
Heparinization
The approach to anticoagulation during pediatric CPB is similar to that for adults. Heparin is administered in a dose of about 4 mg/kg (400 U/kg) prior to initiation of bypass, either directly into the right atrium or into a central venous catheter; teams at some centers include a portion of the total heparin dose in the CPB pump prime. Confirmation of heparin injection by blood aspiration, as well as the adequacy of heparin effect, should be performed before beginning CPB. The activated clotting time (ACT) is the primary method of monitoring the efficacy of heparin anticoagulation (and reversal) in infants and children. Typical guidelines require an ACT of greater than 400 seconds before initiating CPB, and ACT should be maintained between 400 and 600 seconds during CPB to prevent activation of blood coagulation pathways and clot formation. Inadequate concentrations of heparin are believed to be a major contributor to excessive activation of the coagulation and fibrinolytic systems.59 Other methods of monitoring heparin effect, anticoagulation, and clotting parameters, such as blood heparin concentration and thromboelastography, are adjunctive at present and used mainly to assess residual heparin activity and diagnose and treat coagulopathies after termination of CPB.60
Although substantive data are scant, there is some evidence that, generally, neonates and young infants are more sensitive to the effects of heparin administered for CPB, and that the efficacy and duration of heparin-based anticoagulation is significantly more variable in neonates and young infants.59,61–63 Potential mechanisms include the variable and generally lower levels of both procoagulant and anticoagulant factors present during the first few months of life and in some patients with congenital heart disease.64–67 The degree of hypothermia, amount of hemodilution, and relative immaturity of drug metabolism may also contribute to increased and prolonged heparin effect in infants. Heparin resistance, on the other hand, is seen infrequently in infants, although sporadic examples resulting from recent heparin exposure or antithrombin III deficiency do occur. Heparin-induced thrombocytopenia and thrombosis appear to be less common in infants and children than in adult heart surgery patients, but the incidence may go up as the number of children who are repeatedly exposed in the operating room, catheterization laboratory, and other sites continues to increase.68,69 There is at present only limited and anecdotal experience with the use of heparin alternatives such as hirudin and argatroban for anticoagulation during pediatric CPB.
Heparin Reversal
Protamine sulfate is administered at the end of CPB to reverse the anticoagulant effects of heparin. As with heparin, there is little prospective, controlled information about the dosing of protamine in neonates and infants. Protamine dosing is usually based on body weight (3 to 4 mg/kg) or in a ratio to the heparin dose (milligrams-to-milligrams) of 1:1 or 1.3:1; in vitro titration to neutralize heparin in a patient sample is also employed by some. In general, the target ACT after protamine administration is within about 10% of the pre-CPB baseline. The first two empirical methods usually result in relative protamine excess, which is intentional because of greater heparin sensitivity and duration in infants, and because of other factors that may potentiate heparinization, such as hemodilution, hypothermia, and delayed metabolic clearance. However, as with adults, there is evidence that empirical protamine dosing is associated with excess protamine administration and perhaps greater blood loss and transfusion requirements as compared with doses that directly measure blood heparin using titration or other methods.62,63,70 Another argument against empiric dosing is that relatively small excesses of circulating protamine compared with heparin may have direct antiplatelet effects that can exacerbate bleeding after CPB.71
Typically, the drug is administered slowly over approximately 10 minutes. For unclear reasons, severe hypotensive, pulmonary vasoconstrictive, or anaphylactic/anaphylactoid reactions to protamine are uncommon in infants and children.72 Of these, hypotension is most frequent, occurring in between about 1.5% and 3% of protamine administrations. It is dependent on dose and rate of administration, most likely resulting from histamine release, usually fairly mild and transient, and responsive to volume replacement or calcium administration. Severe pulmonary vasoconstriction appears to be much less common than in adults, may be due to complement activation or pulmonary thromboxane release, and can be particularly troubling in patients with depressed contractile function.
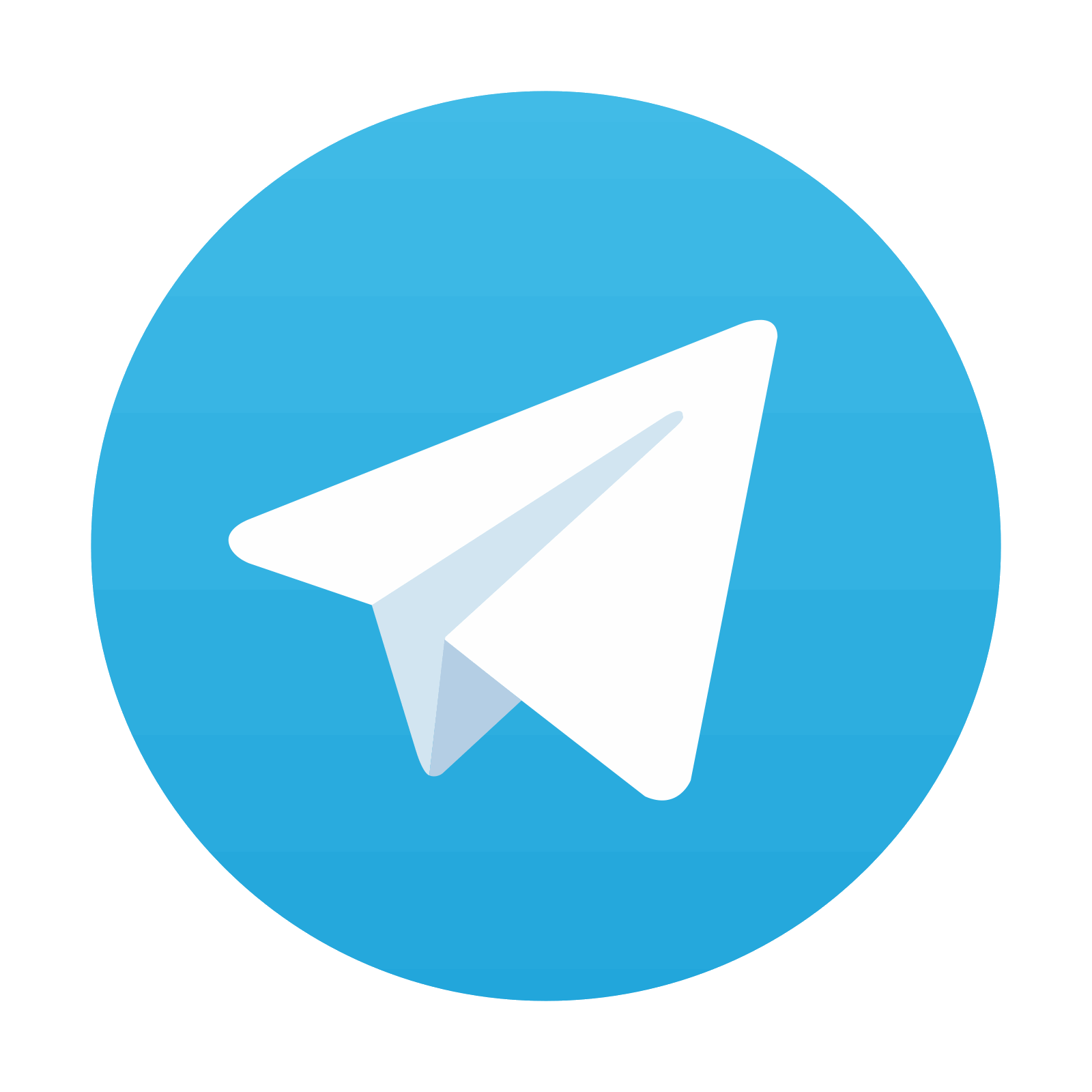
Stay updated, free articles. Join our Telegram channel
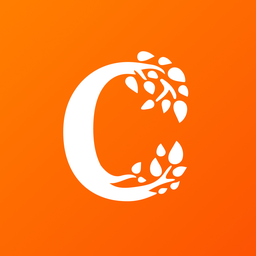
Full access? Get Clinical Tree
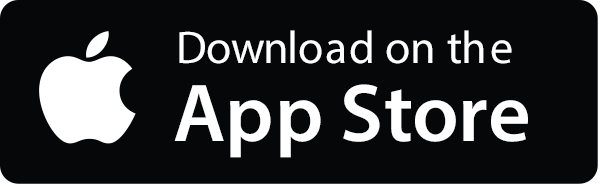
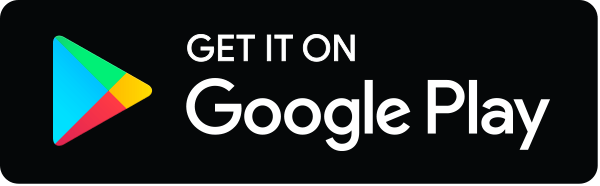