35 Atrial arrhythmias are the most common sustained cardiac arrhythmias in humans. Atrial arrhythmias, mainly atrial fibrillation (AF), which often provoke disabling symptoms and severe complications,1 are considered major causes of morbidity and mortality. Additionally, it has been observed that AF induces changes in the atrial myocardium that help to perpetuate the arrhythmia “AF begets AF.” These changes, called atrial remodeling, include alterations in the expression of various ion channels affecting electrical activity of the atrial cells and changes in anatomical structure. They have been described in animals2 and in humans.3,4 Changes in the electrical activity cause a decreased effective refractory period (ERP) and slowed conduction and reduction in rate adaptation of ERP,2,3 which may help the initiation and persistence of AF, as suggested by experimental studies.2,3 Despite significant advances in our knowledge about the mechanisms that lead to the onset and maintenance of atrial arrhythmias, they remain incompletely elucidated. It is thought that atrial arrhythmias can be caused by focal ectopic activity, localized reentry, or multiple propagating wavelets.4–9 It is important to understand the mechanisms of initiation and perpetuation of atrial arrhythmias because they have a strong influence in the design of antiarrhythmic therapies. Several experimental and clinical studies have shown that different mechanisms lead to differences in the characteristics of spatiotemporal organization of atrial arrhythmias. Studies of the spatiotemporal organization of atrial arrhythmias are currently being performed by analyzing the electrograms (EGM) recorded at different points on the atrial surface using different signal analysis techniques, including analysis of EGM morphology,10,11 dominant frequency (DF),7,12,13 and regularity12 or organization indexes (OI).14 In recent high-density mapping studies, areas of complex fractionated atrial electrograms (CFAE) and high DF have been proposed as critical regions for the maintenance of AF6,8,15 and have become target sites for AF ablation. However, ablation of high-DF areas has proven to be incompletely effective in patients with persistent AF.12 In addition, it is not universally accepted that improvement in AF ablation occurs after CFAE ablation in patients with persistent AF.16 In recent years, computational modeling has provided a framework of multiscale integrated models for the study of cardiac arrhythmias.17,18 Computational cardiac models that simulate atrial activity have proved to be an important tool in facilitating understanding of the complex mechanisms underlying atrial arrhythmias. Computer simulations of atrial tissue have provided hypotheses that have been tested experimentally and, additionally, have been used to investigate and to explain experimental and clinical observations. This chapter reviews the insights provided by these atrial models, with emphasis on the contributions of three-dimensional (3D) atrial models, and shows various examples of atrial arrhythmias simulated using a realistic 3D model of human atria developed by our group. Several computer atrial models have been developed and used to study atrial arrhythmias and to evaluate the efficacy of different therapeutic approaches. The first was developed by Moe et al19 Using a cellular automata model, they suggested that AF can result from the activity of multiple independent wave fronts propagating simultaneously throughout the entire atria. The multiple wavelet hypothesis, as a mechanism underlying AF, was confirmed experimentally after several years.4 The development of comprehensive mathematical models of the electrophysiological activity of human atrial cells20–23 has provided a useful tool for investigating the contribution of different ionic currents to atrial arrhythmias. Dr. Jalife’s group have been pioneers in combining experiments with simulation studies to understand the origin and maintenance of AF. Using a simplified 2D model of human atrial tissue, they have shown the important role of the IK1 current in stabilizing rotors during chronic AF, and how the blockade of IKur or Ito can terminate rotor activity.24 On the other hand, a recent study using a 2D model of atrial tissue suggested that electrotonic interaction between myocytes and fibroblasts plays an important role in the genesis of CFAE.25 The first 3D model of the human atria, which combined a detailed cellular ionic model (Nygren cell model20) with realistic geometry, was presented by Harrild and Henriquez.26 They showed how bundles of atrial muscle including the crista terminalis (CT), the pectinate muscles (PM), Bachmannn’s bundle (BB), and the limbus of the fossa ovalis play an important role in determining spread of the propagation wave front. Vigmond et al27 presented a less geometrically realistic model but included morphologic details such as electrical interatrial communications, anisotropic conduction, and muscular structures. They observed how specific structures in the atria—coronary sinus (CS), CT, PM, and orifices such as inferior caval vein (ICV) and superior caval vein (SCV)—play an important role in reentrant activity. Lausanne’s group introduced a simplified fiber structure in a 3D model for studying the impact of anisotropy on the morphology of electrograms10 and later evaluated the effects of different ablation patterns in the treatment of AF,28 showing that the prediction of converting AF to sinus rhythm observed experimentally was very similar to what was predicted by the computer model.29 This model, in combination with clinical data, has also been used to show that atrial fibrillatory cycle length is an important predictor of AF duration and of the rate of AF termination by ablation.30 In 2006, Seeman et al used data extracted from a visible female dataset to develop a 3D model of the atria with great anatomical detail. They included anisotropic properties of the tissue and heterogeneous electrophysiological properties to study the contributions of different anatomical structures in normal atrial conduction.31 Aslanidi et al presented a multiscale computational model based on this 3D model that included a human torso model to study the mechanisms underlying atrial arrhythmias32 and to test the treatment efficacy of antiarrhythmic drugs.33 The effect of electrical versus structural remodeling on AF perpetuation has been studied using a 3D model based on Harrild and Henriquez’s work.26 It has been observed that both electrical remodeling and structural remodeling contribute to APD shortenings, whereas structural remodeling is the main contributor to reduced conduction velocity.34 All of the described 3D atrial models used a simplistic fiber structure lacking detailed description of fiber direction in the atria. In 2009, our group developed a 3D atrial model that integrated realistic geometry and structure, as well as heterogeneous electrical properties, with detailed fiber orientation in the whole atria.35–39 In recent reports,40,41 semiautomatic methods were used to incorporate atrial anisotropy (including fiber orientation) and heterogeneities into patient-specific 3D models based on geometries obtained from magnetic resonance imaging (MRI) data, gaining an important step toward the use of specific atrial models in the clinic. A realistic 3D model of human atria that includes fiber orientation was previously developed by our group.35–37 The original set of surfaces of the model was based on the work of Harrild and Henriquez.26 These surfaces were modified in accordance with data from the literature and histologic observations.42,43 The model comprises the main structures (Figure 35-1): left and right atrial chambers (LA and RA), 20 pectinate muscles (PM), the fossa ovalis (FO), Bachmann’s bundle (BB), the crista terminalis (CT), left and right appendages (LAPG and RAPG), left and right pulmonary veins (LPV and RPV), superior and inferior caval veins (SCV and ICV), the isthmus of RA, atrioventricular rings (AVR) and the coronary sinus (CS). The sinoatrial node (SAN) is situated near the ostium of the SCV. Figure 35-1 Frontal and dorsal views of the human atrial model. Colors represent areas with different fiber orientation. Arrows indicate the orientation of the fibers in the main areas of the atria. AVR, Atrioventricular rings; BB, Bachmann’s bundle; CS, coronary sinus; CT, crista terminalis; FO, fossa ovalis; ICV and SCV, inferior and superior caval veins; LA and RA, left and right atria; LAPG and RAPG, left and right appendages; PM, pectinate muscles; RPV and LPV, right and left pulmonary veins; SAN, sinoatrial node. (From Tobón, C, Ruiz-Villa C, Heidenreich E. et al: Three dimensional human atrial model with fiber orientation. Electrograms and arrhythmic activation patterns relationship. Plos One 8(2):1–13, 2013, Fig. 1.) Several experimental observations have been made about the role of anatomical structure and electrophysiological heterogeneity in atrial electrical activity in both physiological and pathologic conditions.42,44 Notably, this atrial model includes a realistic fiber orientation based on histologic observations.42,45 The model was divided into 42 areas (see the colored zones in Figure 35-1), and a realistic fiber direction was assigned to each region. In Figure 35-1, the main areas of the model and their fiber orientation (indicated by arrows) are shown. It is noted that circulating muscle bundles42,46 around the CS, the LPV and RPV, the SCV and ICV, the AVR, and both appendages (RAPG and LAPG) are present, whereas BB, CT, and PM show aligned fibers along their longitudinal axes. The posterior walls of both atria (RA and LA) consist of mainly vertical fibers, whereas the fibers between the SCV and the ICV have a horizontal direction, provoking a complex arrangement of vertical, horizontal, and circular fibers in the ostium of the pulmonary veins.45 Nygren’s model20 of human atrial action potential (AP) was used to simulate cellular electrical activity. Electrophysiological heterogeneity was included to reproduce AP in different zones of the atria47: PM, CT, AVR, left and right appendages (APG), and atrial working myocardium (AWM), which includes the remaining atrial structures. To obtain these AP models, maximum conductance of It, IKr, and ICaL was modified.35 To reproduce electrical remodeling conditions, changes in conductance and kinetics of different ionic channels observed in experimental studies of permanent AF3,48 have been incorporated into these AP models. Maximum conductance for IK1 was increased by 250% and for ICaL and It was decreased by 74% and 85%, respectively; kinetics of the fast inactivation of ICaL was increased by 62%, the activation curve of It was shifted by +16 mV; and the inactivation curve of INa was shifted by +1.6 mV.49 Figure 35-2 shows the last AP obtained when a train of 10 stimuli at a basic cycle length of 1000 ms was applied for the different atrial cellular models considered (AWM, PM, CT, APG, and AVR) under both physiological (control) (Figure 35-2, A) and remodeling conditions (Figure 35-2, B). The APD90 values (see table in Figure 35-2, C) show that under control conditions, APD90 ranged from 180 ms to 307 ms. It is important to note, however, that atrial remodeling decreased the APD90 values in the whole atria (ranging from 56 ms to 92 ms) as well as the APD dispersion. Figure 35-2 AP for the different types of atrial cells: crista terminalis (CT), pectinate muscles (PM), left and right appendages (APG), atrioventricular rings (AVR), and atria working myocardium (AWM), which includes the rest of the tissue; under (A) physiological conditions (control) and (B) remodeling conditions (remodeled), at a BCL = 1000 ms. C, APD90 values. D, Restitution curves for physiological and remodeling conditions for cells of the AWM. (From Tobón, C, Ruiz-Villa C, Heidenreich E. et al: Three dimensional human atrial model with fiber orientation. Electrograms and arrhythmic activation patterns relationship. Plos One 8(2):1–13, 2013, Fig. 3.) Figure 35-2, D illustrates restitution curves for AWM, for both physiological (control) and electrically remodeled cells. It is possible to observe how the remodeling condition provokes a reduction in APD and in ADP rate adaptation, inducing a decrease in ERP and a reduction in rate adaptation of ERP, which will facilitate ectopic beat propagation. where Sv corresponds to the surface-to-volume ratio, D is the conductivity tensor, Cm is the specific membrane capacitance (50 pF), Iion is the total ionic current that crosses the membrane cells, Vm is the membrane potential, and Istim is the stimulus current. The monodomain equation was solved using a finite element method. Conductivity values were assigned to obtain realistic conduction velocities observed in the different atrial zones: 25 cm/s in very slow regions (SAN), 54 cm/s in slow regions (PV), 120 cm/s in fast regions (BB, limbus of the FO and PM), and 143 cm/s in very fast regions (CT bundle), whereas the remaining atria had a conduction velocity of 69 cm/s.50,51 An anisotropic ratio of conductivity was also introduced in agreement with experimental data.51,52 The isthmus of the RA and the SAN were set isotropic, whereas an anisotropic ratio of 1 : 2 was used for BB, limbus of FO, PV, and AWM.52 Finally, an anisotropy ratio of 1 : 9 was used for CT.51
Supraventricular Arrhythmias in a Realistic 3D Model of the Human Atria
Brief Summary of Atrial Computer Models
Building a 3D Model of the Human Atria
Anatomical Characteristics
Electrophysiological Models
Propagation and Atrial Electrograms
Stay updated, free articles. Join our Telegram channel
Full access? Get Clinical Tree
Supraventricular Arrhythmias in a Realistic 3D Model of the Human Atria
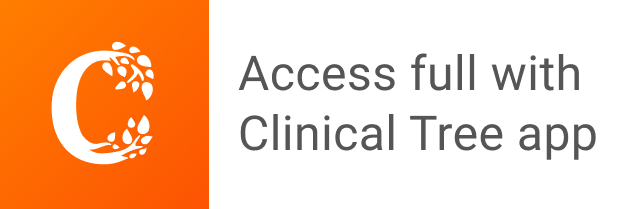