(1)
The Molecular Cardiology and Neuromuscular Institute, Highland Park, NJ, USA
Abstract
Stem cells are undifferentiated cells characterized by the presence of the potential for self-renewal and the unique ability to differentiate into various cell types—pluripotency. Various cell types proposed as candidates for cardiac cell-based therapy can be divided into two groups: allogenic and autologous according to their origin. Allogenic cells include embryonic stem cells (ESCs), fetal cardiomyocytes, and umbilical cord-derived cells, whereas autologous or adult stem cells include bone-marrow- and adipose-derived stem cells, skeletal myoblasts, resident cardiac progenitor cells, and induced pluripotent stem cells (iPSCs). Different cell types and delivery strategies have been examined in experimental and clinical settings; however, neither the ideal cell type nor cell delivery method for cardiac cell therapy has yet emerged.
Emerging evidence suggests that mitochondria play an essential role in ESC maintenance and differentiation. Undifferentiated ESCs and iPSCs contain the decreased number of immature mitochondria displaying perinuclear localization. Levels of mtDNA and factors, implicated in mtDNA maintenance, are also significantly diminished. Early embryos, undifferentiated ESCs, and iPSCs typically exhibit prevalence of anaerobic glycolysis as the major energy source. Expression of glycolytic enzymes is upregulated, whereas expression of subunits of ETC is downregulated resulting in lower O2 consumption and lower levels of intracellular ATP. Upon ESC differentiation into cardiomyocytes, the expression of glycolytic enzymes is downregulated, while the expression of OXPHOS subunits and citric acid cycle enzymes is upregulated. Hence O2 consumption, OXPHOS, and ATP generation are significantly increased, mtDNA replication and mitochondrial biogenesis are resumed, and mature mitochondrial networks are formed.
Switch in energy metabolism from glycolysis to OXPHOS during differentiation of ESCs can lead to increase in intracellular levels of reactive oxygen species (ROS). The upregulation of the antioxidant system in differentiating ESCs does not compensate the increased generation of ROS. Accumulation of oxidative damages in mtDNA in differentiating ESCs leads to mutations in mitochondrial genomes affecting ATP production, Ca2+ homeostasis, cell proliferation and differentiation resulting subsequently in apoptosis. ROS also play an important role as a critical signaling intermediate implicated in control of ESC proliferation and differentiation. ROS-induced deacetylase silent information regulator 1 (SIRT1) activation contributes to ESC maintenance by both inducing mitochondria-mediated apoptosis of damaged cells and by attenuating p53-mediated inhibition of the pluripotent factor Nanog. The p53-telomere axis appears to be also involved in this complex regulatory circuitry. Further insights gleaned from study of mitochondrial function in stem cells will no doubt accelerate the design of realistic clinical cell-based therapy for cardiovascular disorders.
Introduction
Myocardial infarction (MI) and heart failure (HF) are the leading causes of death in the western world, affecting, for example, only in the United States more than eight and five million people, respectively [1–3]. Despite a significant improvement of death rates during the last two decades, these dramatic statistics show the limits of current interventional and pharmacological therapies for cardiac disease and underscore the urgent need for new therapeutic strategies.
Over the past two decades, stem-cell-based therapy has emerged as a promising alternative approach for the treatment of patients with cardiovascular pathologies. Emerging evidence from experimental animals and clinical trials, involving thousands of patients, suggests that stem cell transplantation into the damaged heart could induce cardiac muscle regeneration and improve myocardial function [2, 4, 5]. The ultimate goal of this revolutionary strategy is to repair injured myocardium to enhance the cardiac function and prevent MI. To achieve this aim multiple limitations must be overcome, including the difficulties of producing a sufficient number of cells and their low survival after transplantation, as well as the problems of the efficient integration of the engrafted cells into the injured myocardium and modest and transient improvement of cardiac function.
The field of cardiovascular stem cell research and regenerative cardiovascular medicine has rapidly progress since the original report of embryonic stem cells derived from human blastocysts in 1998 [6]. However, most stem cell research has focused on the role of the nuclear genes in the pluripotent status of stem cells and in the control of their differentiation into the desired specific cell types. While the mitochondria play a critical and multifaceted role in the high-energy-demanding myocardium, information concerning mitochondrial structure, function, and dynamics in progenitor and stem cells, proposed for cardiac muscle repair, is currently limited. The lack of protective histones, close proximity to the OXPHOS, a potent source of reactive oxygen species (ROS), and less efficient DNA repair system lead to a significantly higher mutation rate in mitochondrial DNA (mtDNA) compared to that of nuclear DNA (nDNA) [7]. It is well established that point mutations and deletions in mtDNA are associated with various diseases, such as cardiovascular and neurodegenerative disorders, diabetes, cancer, and aging; however, how they affect stem cell functions remains largely unknown [8–15]. Moreover, the use of stem cells, regardless of their origin, which contain mtDNA abnormalities, for cell-based therapy can result in the development of tumors. Thus, mitochondrial function and dynamics in progenitor and stem cells represent an important, although yet largely unexplored, topic for stem cell biology and regenerative medicine.
In this chapter, the major stem cell types and delivery methods, proposed for cardiovascular repair therapy, will be overviewed. Then the two key features of stem cells, which underlay their use in cell-based therapy, self-renewal, and pluripotency, will be discussed. Finally, recent studies of mitochondrial metabolism and dynamics in stem cells will be presented.
Stem Cell Types and Delivery Techniques
Stem cells represent undifferentiated cells characterized by the potential for self-renewal and by the unique ability to differentiate into various cell types, so-called pluripotency. Multiple cell types, differing in their origin, morphology, function, and ability to differentiate into specific cell types, have been considered as candidates for cardiovascular repair therapy. Although the ideal cell type for heart cell-based therapy has not yet emerged, it should satisfy the following criteria:
Be safe, that is, not inducing cardiac arrhythmias, undesirable myocardial remodeling, or tumor growth.
Integrate into the host myocardium to restore functional cardiac muscle and improve heart function.
Circumvent the immunocompatibility problems.
Be amenable to efficient delivery into the damaged heart area by safe minimally invasive techniques.
Be a standardized “off-the-shelf” product.
Fulfill ethical and legal requirements.
Various cell types proposed for the cell-based repair of damaged heart can be divided into two groups: allogenic and autologous according to their origin. Allogenic cells include embryonic stem cells (ESCs), fetal cardiomyocytes, and umbilical cord-derived cells, whereas autologous or adult stem cells include bone-marrow- and adipose-derived stem cells, skeletal myoblasts, resident cardiac progenitor cells (CPCs), and induced pluripotent stem cells (iPSCs) (Table 9.1).
Table 9.1
Candidate cell types for cell-based therapy of cardiovascular diseases
Cell Type | Source | Advantages | Disadvantages |
---|---|---|---|
Allogenic cells | |||
Embryonic stem cells | Embryo | Self-renewal Pluripotent Integration with the host cardiomyocytes | Teratoma formation Immunologic rejection Ethical & legal issues |
Fetal cardiomyocytes | Fetal heart | Cardiac origin Integration with the host cardiomyocytes | Immunologic rejection Ethical & legal issues |
Umbilical cord-derived cells | Cord blood | Reduced risk of immunological rejection No ethical issues | Limited testing |
Autologous cells | |||
Bone marrow stem cells | Bone marrow Blood Adipose tissues | Differentiate into cardiomyocytes & vascular cells Paracrine cardiac effects | Pluripotency uncertain No direct myocardial regeneration |
Skeletal myoblasts | Muscle biopsy | Increased resistance to ischemia Low risk of tumorigenesis | Do not differentiate into cardiomyocytes No electrical integration Ventricular arrhythmias |
Resident cardiac progenitor cells | Cardiac biopsy | Differentiate into all cardiac lineages Clinical trials underway | Invasive cardiac biopsy Use of xenogenic antibodies for isolation Limited testing |
Induced pluripotent stem cells | Dermal & other tissues | Readily obtained Differentiate into various cells | Teratoma formation Limited testing |
Allogenic Cell Types
Embryonic Stem Cells and Fetal Cardiomyocytes
ESCs are the prototypical stem cells, which give rise to all cells of three embryonic germ layers. They exhibit indefinite self-renewal capacity and have the highest developmental potential (pluripotency), including the ability to differentiate into cardiomyocytes [16]. Several protocols to differentiate human ESCs into cardiomyocytes have been developed [17]. Importantly, while cardiomyocytes, isolated from differentiating ESCs, exhibit all major features of primary cardiomyocytes, they resemble fetal rather than adult cardiomyocytes [16, 18]. The self-renewal capability of ESCs appears to depend on the upregulation of telomerase and on the suppression of senescence factors p16 and p21, whereas their pluripotency is mediated by intricate network of transcription factors, including Nanog and Oct-4 [19–21].
In animal models, the transplanted ESC-derived cardiomyocytes couple electrically and contract in synchrony with the host cardiomyocytes and form stable grafts for at least 12 weeks [4]. However, improvement in heart function has been observed 4 weeks after transplantation, with no beneficial effects after 12 weeks of transplantation [22, 23].
Fetal cardiomyocytes were one of the first cells proposed for the therapeutic use in heart repair. Similar to ESCs, fetal cardiomyocytes, transplanted into mouse ischemic or failing hearts, form stable grafts and improve cardiac function [24, 25]. However, both ESCs and fetal cardiomyocytes have several potential disadvantages. As allogenic cells, they would require immunosuppression to prevent immune rejection after transplantation [26]. Moreover, the therapeutic use of human ESCs and fetal cardiomyocytes has raised the ethical and legal concerns although progress has been made to circumvent some of these issues. Finally, the propensity of ESCs to form teratomas after transplantation into various tissues, including the heart, raises serious concerns regarding their malignant potential [27–29].
Umbilical Cord-Derived Stem Cells
Cord blood contains a significant amount of non-hemopoietic immunologically naïve stem cells [30]. They typically do not express HLA class II antigens, and therefore, their transplantation reduces the risk of immunological rejection. Human cord blood mononuclear cells (hUCBCs), containing hematopoietic and mesenchymal stem cells, are presently used for treatment of patients with acute leukemia [30]. Importantly, intramyocardial injection of hUCBCs to experimental animals with cardiomyopathy has attenuated fibrosis, reduced infarct size, and improved cardiac function [31, 32].
Autologous Cell Types
Compared to allogenic stem cells, autologous adult stem cells are characterized by a lower capacity to self-renew and to differentiate into various cell types. On the other hand, they exhibit the reduced risk of tumor formation and do not face immunological challenges.
Bone-Marrow-Derived Cells
The bone marrow (BM) contains two main cell subsets, hematopoietic stem cells (HSCs) and mesenchymal stem cells (MSCs); both can be isolated from the peripheral blood or by BM aspiration. HSCs express CD34 and CD113 cell-surface antigens and have high self-renewal ability and serve as precursors of blood cell lineages [33–35]. MSCs express CD44, CD90, CD105, CD106, and CD166 cell-surface markers, while they are negative for hematopoietic (CD14, CD34, and CD45) and endothelial (VEGFR2, CD34, and CD105) markers and can differentiate into several non-hematopoietic cell types [36–38]. MSCs can be isolated not only from BM but also from adipose tissues.
Both HSCs and MSCs are capable to differentiate into cardiomyocytes or generate chimeras via fusion with host cells after injection into failing hearts improving cardiac function [39–44]. MSCs can be isolated, expanded, and maintained in culture easier than HSCs. In addition, due to the lack of major histocompatibility complex (MHC) class II expression, they do not induce immunological rejection [45]. Thus, MSCs represent attractive candidates for cardiac stem cell therapy.
Clinical trials using BM-derived progenitor cells have proved that the procedure is safe; however, they have demonstrated that the injected cells fuse with the host cardiomyocytes rather than transdifferentiate into new cardiomyocytes [40, 41]. Moreover, beneficial effects of BM cell transplantation have been modest [27, 46]. Taken together, no direct myocardial regeneration has currently been documented using autologus BM cells, and modest improvement in cardiac function probably results from poorly understood paracrine effects [47]. Most recently, the largest randomized double-blind, placebo-controlled trial using autologous BM-derived mononuclear cells for therapy in patients with chronic ischemic heart disease and left ventricular dysfunction has not shown improvement in left ventricular end-systolic volume compared to placebo, while a significant improvement in left ventricular ejection fraction has been observed after the treatment [48]. The transplanted BM-derived cells appear to contribute to myocardial repair as a part of inflammatory response that induces angiogenesis, cardiomyocyte survival, and left ventricular remodeling after infarction [5].
Skeletal Myoblasts
Injection of autologous skeletal myoblasts into the ischemic heart was one of the first cell-based heart repair approaches [49, 50]. Skeletal myoblasts have two advantages compared to BM-derived cells: they are more resistant to ischemia and more clearly retain the regeneration capacity. However, despite initial enthusiasm, current evidence demonstrates that injected skeletal myoblasts in animal infarct models can differentiate into myotubes, but not into cardiomyocytes [50, 51]. Postmortem pathology has failed to demonstrate integration of transfected skeletal myoblasts into recipient myocardium [52]. Moreover, although modest beneficial effect on ventricular function has been reported, myotubes have not integrated electrophysiologically with host cardiomyocytes and therefore increasing risk of ventricular arrhythmias [53–55].
Resident Cardiac Progenitor Cells
An alternative to the above-outlined transplantation of exogenous stem cells to replace lost cardiomyocytes would be to induce an endogenous pool of cardiac progenitor cells in the damaged heart. In contrast to humans, amphibian and fish are able to efficiently regenerate a heavily injured heart [56]. In the adult zebra fish, which represent a classical model for regeneration studies, the resection of up to 20% of the ventricle can be fully repaired by the proliferation of preexisting cardiomyocytes [57–60].
While adult mammalian hearts lack such high regenerative ability, they also display some limited self-repair following infarction, albeit at very low level [61]. In contrast to the zebra fish, mouse heart regeneration appears to be predominantly mediated by endogenous progenitor cells rather than by the proliferation of preexisting cardiomyocytes [5].
The adult human heart has traditionally been considered as a terminally differentiated organ. However, it also has some regenerative potential [62–64]. Recent radiocarbon analyses of human postmortem hearts have provided conclusive proof of cardiomyocyte renewal with the age-dependent replacement frequencies ranging from ∼1% per year at age 20 to 0.45% at age 70 [62]. Based on this kinetic, approximately 45% of cardiomyocytes would be renewed over a normal human life span. The human left ventricle contains 2–5 × 109 cardiomyocytes, and the failing heart can lose up to 25% of cardiomyocytes in a few hours [65–68]. Therefore, poor regenerative capacity of the adult human heart is obviously not sufficient to replace massive cardiomyocyte loss in the damaged myocardium.
Emerging evidence suggests that the adult mammalian heart contains a compartment of resident CPCs. Several laboratories using various cell markers, such as c-kit+, Sca-1+, and Isl1+, and different isolation methods have identified a minute pool of resident CPCs in the adult heart [69–75]. These cells are capable of proliferating in culture and differentiating into cardiomyocyte and vascular lineages [76]. While initially it was not clear to what extent CPCs, identified by different laboratories, overlap, subsequent studies have demonstrated that once-distinct CPC populations share markers and can represent the same type of cells at different stages of maturation [77, 78].
Human CPCs can be isolated from the normal heart using a minimally invasive biopsy. Isolated human c-kit+ CPCs are able to expand and differentiate in culture predominantly into cardiomyocytes, smooth muscle, and endothelial cells. Furthermore, these cells, when injected to the infarcted myocardium of immunodeficient or immunosuppressed rodents, integrate functionally with rodent myocardium and appear to improve cardiac function [77, 79]. Some studies have reported that injected c-kit+ CPCs mediate a large-scale repair of the infarcted heart, while others have indicated more modest regenerative effect [79, 80]. Taken together, these findings suggest the possibility of using CPCs in cardiac cell-based therapy. A National-Institutes-of-Health-sponsored clinical trial using purified human c-kit+ CPCs as an adjunctive treatment for patients undergoing bypass surgery is now underway (ClinicalTrials.gov identifier NCT00474461) [5].
One of the disadvantages of c-kit+ CPCs is the use of xenogenic antibodies for their isolation. Another CPC pool, which can be used for cardiac cell-based therapy, is cardiosphere-derived cells (CDCs); their isolation does not require use of antibodies. CDCs can be isolated from cardiac biopsy material based on their ability to form spheroids in suspension cultures [71, 81]. They represent a heterogeneous mixture of cells, including c-kit+-expressing cells, capable to differentiate into cardiomyocytes in vitro and in vivo and improve cardiac function when injected into the infarcted zone in animal models [71, 81, 82]. A clinical trial using autologous CDCs isolated from human heart biopsy specimen and injected intracoronary in patients with MI has recently been initiated (NCT00893360) [5].
Induced Pluripotent Stem Cells
The challenges with ethics and immunocompatibility regarding ESC-based therapy have stimulated the development of a novel strategy relying on the reprogramming of autologous adult differentiated cells, such as dermal fibroblasts, into iPSCs using ectopic expression of specific genes responsible for pluripotency [83–85]. Human iPSCs were initially generated from human skin fibroblasts using retroviral transduction of up to four stem-cell-related transcription factors Oct4, Sox2, c-MYC, and Klf4 [86, 87]. Subsequently this technique has been successfully applied to other cell types obtained from patients [88–91]. While Oct4 and Sox2 are essential for the reprogramming of adult somatic cells into iPSCs, they can be generated in the absence of c-MYC transduction [92–94]. Activation of these transcription factors in adult somatic cells results in their dedifferentiation into pluripotent cells, resembling ESCs. Human iPSCs proliferate in culture, have normal karyotypes, and express characteristic surface markers and telomerase.
It has recently been shown that iPSCs can be directed to differentiate into cardiovascular and hematopoietic progenitor cells [95–98]. Recent advances in cardiac developmental biology have led to designing protocols to enhance cardiogenic differentiation of ESCs and iPSCs yielding highly enriched pools of cardiomyocytes. These approaches exploit modulations of cardioinductive factors, such as activin A, the bone morphogenic protein 4 (BMP4), and the Wnt/β-catenin signaling [22, 99–102]. Importantly, small molecules may significantly enhance the efficacy of reprogramming [103]. A related strategy is the reprogramming of cardiac fibroblasts, including the scar-forming fibroblasts in the infarcted myocardium, into cardiomyocytes. Two recent reports have provided first encouraging data of using this approach in animal models [104, 105].
While the first-generation iPSCs have involved virus-mediated delivery of reprogramming factors, raising concerns about tumor development, more recently virus-free delivery systems, including direct delivery of reprogramming proteins, excisable transgenes, and synthetic messenger RNA, have been proposed [106–110]. Further carefully conducted studies are required to determine features of iPSCs produced by these novel methods.
In summary, iPSCs have great therapeutic potential; they circumvent the ethical issues and immunocompatibility problems; however, cells generated by current technologies can produce teratomas after transplantation. This serious limitation has to be overcome prior to initiation of clinical trials.
Cell Purification, Delivery, and Engrafting Strategies
Purification of cardiac progenitor cells from a heterogeneous population of differentiating ESCs or iPSCs followed by their expansion provide a significant advantage given the large amount of cells, which are to be replaced in the damaged heart. It is also critical to eliminate from the cellular source, prepared for transplantation, more primitive cells, which can give rise to teratomas. In experimental studies, this can be efficiently achieved through transgenic labeling followed by drug selection or via cell-sorting isolation of the desired cells. However, such approaches are incompatible with requirements for cellular sources designed for clinical trials. Alternative approach is the use of antibody-based purification of desired cell type, for example, human c-kit+ CPCs. Therefore, the identification of additional cell-surface markers of human stem and progenitor cells and generation of corresponding highly specific antibodies will advance cell-based heart repair to the clinical setting.
The ability to produce a sufficient number of cells for cardiac cell-based therapy represents another challenge. Given the failing heart loses up to one-quarter of cardiomyocytes and inefficiency of current cell delivery techniques with 50–90% cell loss, it will be necessary to generate and introduce at least 1010–1011 stem or progenitor cells for a single patient [111, 112]. Thus, the large-scale nature of cell-based myocardial therapy should not be underestimated.
Just the choice of the appropriate cell type alone is not enough for the development of clinically relevant heart repair. Defining the optimal route of delivery of chosen cells is critical for effective cell-based therapy. The constantly contracting heart creates additional difficulties to deliver cells into localized damaged region and immobilize them there.
Several routes of cell delivery, such as intracoronary and intravenous infusion, direct epicardial injection, catheter-based transendocardial injection, and transvenous injection into coronary veins, have been proposed [113, 114]. Intracoronary infusion is the most common cell delivery technique in clinical setting. This method closely resembles that exploited for coronary angioplasty. Clinical trials have confirmed the safety of this route of cell delivery [115]. The major disadvantages of intracoronary infusion are an elevated incidence of coronary events and low efficiency as a significant amount of transplanted cells fails to home in the infarcted myocardium [116, 117]. Although peripheral intravenous infusion has been frequently exploited in experimental animals, presently only one human study has been reported [118]. Transendocardial injection delivers implanted cells to the border zone of the infarcted heart; guided by the mapping system improves the efficiency of this method. Clinical trials using this cell delivery technique are underway.
Regardless of the cell type and delivery technique, all current cell-based cardiac repair strategies suffer from disappointingly low efficiency: cell retention within 24 h of transplantation is <10%, and typically no transplanted cells can be detected after 1 month of delivery [111, 112, 119, 120]. Persistent ischemia due to the hypovascularity of the target damaged region, apoptosis and necrosis due to the loss of survival signals, and the inflammatory milieu of infarcted myocardium and immunologic rejection, if allogenic cells have been used, significantly limit engrafted cell survival [121]. Approximately 90% of the retained cells die within a week, and <1% of them is identifiable 4 weeks after transplantation [122, 123].
Several strategies have been suggested to improve transplanted cell retention, survival, and persistence [22, 124–126]. In the injection-based procedures, transplanted cells can be incorporated into hydrogels, which polymerize in the targeted area and improve cell retention [127]. In a surgical setting, when the myocardium can be directly reached, the damaged area can be covered with biocompatible (e.g., collagen-based) scaffolds seeded with transplanted cells [128–130]. Importantly, the use of scaffolds appears to improve not only retention but also survival of the transplanted cells [131].
Ischemic issue could be ameliorated by the interventional or surgical revascularization of the transplanted region, by inducing the expression of angiogenic factors or by the co-delivery of cells with the angiogenic potential, such as MSCs [128]. Preconditioning of MSCs with the chemokine stromal cell-derived factor 1 (SDF-1) prior to delivery into the rat’s infarcted heart has significantly inhibited apoptosis and enhanced vascularization leading to improved cardiac function [132]. Similar therapeutic effects have been demonstrated with hypoxia-preconditioned MSCs in the rat infarct model [133].
More complex strategy to improve stem cell survival and angiogenesis would be to induce expression of transgenes encoding anti-apoptotic and angiogenic factors and growth hormones. It has been demonstrated that insulin-like growth factor 1(IGF-1)-overexpressing MSCs display better engraftment and survival associated with significantly reduced infarct size in the rat model [134].
Finally, if allogenic cells are used for cell-based cardiac repair, the risk of rejection by the host immune response could be circumvented by careful immune typing of the cells prior to transplantation and by advanced drug immunosuppressive therapy.
Mechanisms of Stem Cell Self-Renewaland Pluripotency
The use of stem cells in cell-based therapy relies on the two key features, which define “stemness”: self-renewal and pluripotency. Self-renewal defines the ability to proliferate indefinitely without lineage commitment, whereas pluripotency defines the capability of undifferentiated cell to generate a variety of differentiated cell lineages [19, 85, 135]. These central characteristics of stem cells are highly regulated by multiple genetic and epigenetic factors. In this section, current insights into the molecular mechanisms underlying this intricate regulatory circuitry will be discussed.
Cell Cycle Regulation and Self-Renewal of Stem Cells
The complex process of self-renewal relies on the balance between cell cycle progression and lineage commitment. ESCs proliferate rapidly and have an abbreviated cell cycle with generation times of ∼8–11 h in murine ESCs (mESCs) and 15–16 h in human ESCs (hESCs); a truncation of G1 is responsible for the shortened cell cycle [136–140].
In most somatic cells, mitogen-activated protein kinase (MAPK) signaling is required for the progression through the early G1 phase [141, 142]. MAPK signaling not only promote cell cycle progression but can also induce differentiation, interlinking both processes [143, 144]. The mitogen-induced accumulation of cyclin D mediates the transition through early G1 of the cell cycle. Cyclin D-CDK4 or cyclin D-CDK6 complexes promote the hyperphosphorylation and inactivation of the retinoblastoma tumor suppressor protein (Rb) and induce expression of cyclin E (Fig. 9.1). Rb inactivation leads to activation of cyclin E-CDK2 complexes, peaking at the G1- to S-phase transition. In contrast to somatic cells, ESCs express low levels of D-type cyclins; therefore, Rb is hyperphosphorylated and inactive throughout the cell cycle, while cyclin E-CDK2 complexes are constitutively active [136, 137]. In addition to high levels of cyclin E-CDK2, ESCs lack CDK inhibitors p21Cip1/Waf1, p27Kip1, and INK family member p16Ink4a [138, 145]. Hence ESCs are characterized by the absence of the proliferative gateway, the “no return point,” called the restriction (R) point, so they progress from M phase directly to the late G1 phase. Thus, ESCs lack the major window for cell-fate decision to enter the cell cycle or to undergo differentiation, allowing thereby for self-renewal [146].
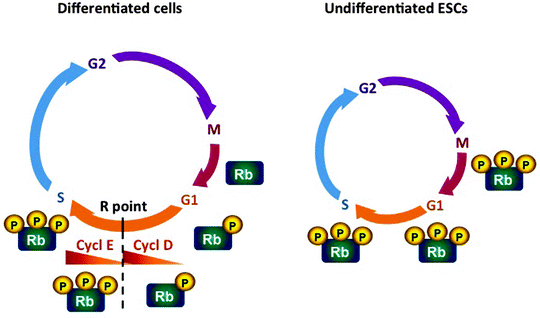
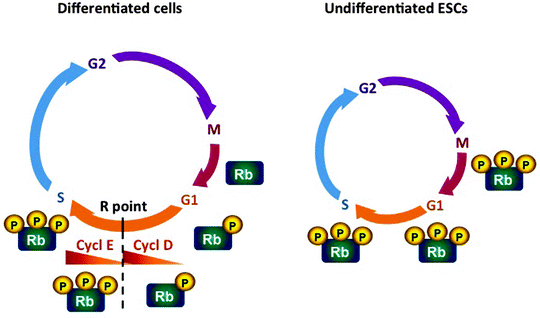
Fig. 9.1
Scheme of the cell cycle in somatic cells and undifferentiated embryonic stem cells. In most somatic cells, the mitogen-induced accumulation of cyclin D mediates the transition through early G1 of the cell cycle. Cyclin D-CDK4/6 complexes (Cycl D) promote the hyperphosphorylation and inactivation of the retinoblastoma tumor suppressor protein (Rb) and induce expression of cyclin E (Cycl E). Rb inactivation leads to activation of cyclin E-CDK2 complexes, peaking at the G1- to S-phase transition. In embryonic stem cells (ESCs), a truncation of the G1 phase is responsible for the shortened cell cycle compared to somatic cells. In contrast to somatic cells, ESCs express low levels of D-type cyclins; therefore, Rb is hyperphosphorylated and inactive throughout the cell cycle, while cyclin E-CDK2 complexes are constitutively active. ESCs lack the restriction (R) point, the major window for cell-fate decision to enter the cell cycle or to undergo differentiation, allowing thereby for self-renewal. CDK cyclin-dependent kinase
While cell cycle of mESCs has been extensively studied, the cell cycle regulation of hESCs is less well characterized. However, the main theme of mitogen-independent cell cycle transition, constitutive expression of cyclin E and Rb hyperphosphorylation, leading to the truncated early G1 phase, is common in both mESCs and hESCs [147].
Molecular Circuit of Pluripotency
“Trinity” of homeodomain transcription factors, Oct4 and Nanog, and the HMG-box transcription factor Sox2, together with Myc, mediate the core transcription regulatory network governing ESC pluripotency in vitro and in vivo [85, 92, 135, 148–150]. Oct4, Sox2, and Nanog are essential for early embryonic development, and deficiency of either of these transcription factors causes early embryonic lethality in mice [151–154]. Oct 4 and Sox2 form a heterodimer, which binds to the Nanog promoter and governs its expression [155, 156]. A large multiprotein Oct4-/Nanog-containing complex has been immunoprecipitated, suggesting the existence of additional proteins that may be implicated in the maintenance of pluripotency in ESCs [157].
The three factors Oct4, Sox2, and Nanog interact together with their own promoters creating autoregulatory loops [158, 159]. Interconnected autoregulatory loops appear to contribute to the stability of gene expression facilitating thereby the maintenance of pluripotency [160, 161]. Consistently, downregulation of Oct4 or Nanog leads to the loss of pluripotency in ESCs and induces their differentiation [153, 154, 162, 163].
The Myc family of basic helix-loop-helix transcription factors with c-, N-, and L-Myc members plays multiple roles in regulation of proliferation, cell-fate determination, cell immortalization, and tumorigenesis [164]. The Myc family members appear not to act as typical transcription factors but rather as epigenetic factors affecting the expression of variety of genes in context-dependent fashion [165, 166]. A role of Myc in mESC self-renewal and pluripotency has first been suggested, based on findings that Myc is significantly downregulated at early differentiation stages and that its ectopic expression makes the maintenance of mESCs independent on the cytokine leukemia inhibitory factor (LIF) [167]. Moreover, Myc expression is essential for a metastable pluripotent state of mESCs [168]. Finally, it has recently been reported that Myc may control the expression of ESC-specific microRNAs (miRNAs) implicated in the regulation of the G1-S transition and maintenance of pluripotency [169–171].
miRNAs represent small (∼22 nt) noncoding RNAs, which are involved in the regulation of gene expression through two principal mechanisms: degradation of mRNAs and inhibition of mRNA translation [172]. miRNAs appear to play an important role in the regulatory circuitry in ESCs; however, the precise mechanisms underlying their action are largely unknown [173–176]. It has been demonstrated that a specific set of miRNAs are preferentially expressed in embryonic tissues and in ESCs [177–179]. Importantly, loss of miRNA-processing enzymes in ESCs is associated with impaired self-renewal and differentiation [173–175]. A critical question how miRNAs interact with transcription factor network to regulate self-renewal and pluripotency of ESCs remains yet unanswered.
The key role of Oct4, Sox2, Nanog, and c-Myc in the regulation of self-renewal and pluripotency of ESCs has further been confirmed by reprogramming embryonic and adult fibroblasts into iPSCs using the mixture of these factors together with Klf4 [83, 180]. Klf4 is the zinc finger transcription factor with oncogenic potential [181]. Importantly, Klf4 facilitates the activation of Oct4 and Sox2 and cooperates with them to activate the Lefty1 core promoter in ESCs [182]. Moreover, Klf4 overexpression inhibits differentiation of mESCs [183]. The role of both oncogenes c-Myc and Klf4 in reprogramming is less characterized, since iPCSs can be generated in the absence of c-Myc, albeit with significantly lower efficiency [87, 93, 94].
The action of transcription factors also relies on the accessibility of the specific genomic sites, containing the target genes [184]. Mouse and human ESCs are characterized by the specific chromatin signatures determined by both genetic and epigenetic mechanisms. ESCs contain nuclei significantly larger than those in differentiated cells, and their chromatin exists predominantly as more open transcriptionally active euchromatin, bearing specific histone complement [185–188].
In summary, pluripotency of ESCs is mediated by a highly complex interplay of signal transduction pathways, specific transcription factors, chromatin-remodeling systems, and regulatory RNAs (Fig. 9.2) [85, 149, 150]. c-Myc contributes to various aspects of the cell cycle progression and pluripotency. It acts as a licensing factor mediating the relax chromatin structure, activating DNA replication, and facilitating access of the Oct4/Sox2/Nanog trio to the target genes. Various chromatin-remodeling and histone-modifying enzymes are implicated in this process. Oct4, Sox2, and Nanog in cooperation with Klf4 coordinately control expression of several hundred genes encoding other transcription factors. Interestingly, these master regulators contribute not only to activation but also to repression of a specific subset of transcription factors, essential for lineage commitment and differentiation [158, 159]. Epigenetic factors, such as the Polycomb group (PcG) proteins, cooperate with the Oct4/Sox2/Nanog trio in silencing of specific genes facilitating the maintenance of the pluripotent state of ESCs [189–192]. However, the mechanisms of both communication between PcG proteins and the master regulators and their targeting to specific genes, which encode developmental regulators, remain to be elucidated.
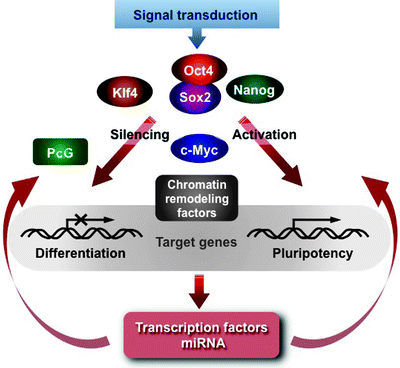
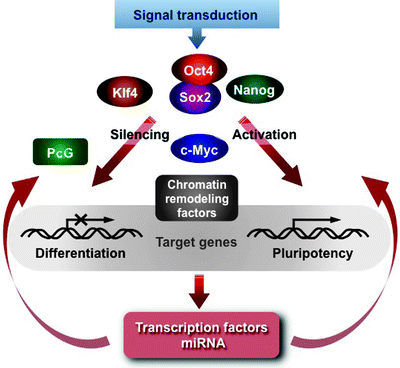
Fig. 9.2
Schematic representation of the pluripotency regulatory circuitry. Signal transduction pathways in response to environment stimuli activate the Oct4/Sox2/Nanog transcription trio, which in cooperation with Krüppel-like transcription factor 4 (Klf4) control expression of several hundred genes encoding other transcription factors. Transcription factor “cellular myelocytomatosis viral-related oncogene” (c-Myc) acts as a licensing factor mediating the relax chromatin structure, activating DNA replication, and facilitating access of the Oct4/Sox2/Nanog/Klf4 to the target genes. Various chromatin-remodeling factors are implicated in this process. Epigenetic factors, such as the Polycomb group (PcG) proteins, cooperate with the Oct4/Sox2/Nanog trio in silencing of specific genes, implicated in differentiation, facilitating the maintenance of the pluripotent state of embryonic stem cells. Other abbreviations: miRNA, microRNA; Nanog, transcription factor “Tír na nÓg legend”; Oct4, octamer-binding transcription factor 4; Sox2, transcription factor “(sex-determining region Y)-box 2”
Mitochondrial Metabolism in Stem Cells
Mitochondria Number and Morphology
Electron microscopic studies have demonstrated that mouse and human early stage embryos and ESCs, derived from the blastocyst, have fewer and less interconnected mitochondria with poorly developed cristae compared to differentiated cells [193–196]. Fewer number of mitochondria in ESCs correlates with lower mtDNA content quantified by real-time PCR. However, when ESCs have been induced for differentiation, increased number of larger interconnected mitochondria with developed cristae have been observed [195, 197]. Moreover, mitochondria in early embryo and ESCs display perinuclear localization [197–200]. This pattern of mitochondrial localization in ESCs closely resembles the mitochondria clustering around the nuclei in fertilized oocytes and early stage embryo of various mammalian species, including humans [201–205]. Importantly, perinuclear localization of mitochondria have also been observed in adult human stem cells, such as HSCs and MSCs [206, 207]. Again, upon differentiation perinuclear arrangement of mitochondria changes to more homogeneously spread in the cytosol. Based on these observations, it has been suggested that the fraction of cells with perinuclear mitochondrial localization in stem cell pools might serve as an indicator of their “stemness.” [198]
Mitochondrial DNA Dynamics
Primordial germ cells and undifferentiated ESCs contain a low number of mtDNA molecules, which is significantly increased during differentiation [208]. A threshold of ∼100,000 mtDNA molecules has been suggested as an indicator of oocyte competence and fertilization outcome [209–211]. The expression of mtDNA replication machinery mirrors these dynamics. Very low expression levels of proteins, implicated in mtDNA replication and transcription, such as POLγA, POLγB, and TFAM, have been demonstrated at the early cleavage stages in various mammalian species [211–213]. Early mtDNA replication has been linked to a global embryonic genome activation [214, 215].
Consistently, ESCs, derived from the inner cell mass (ICM), have a low expression of mtDNA replication and transcription factors, including POLγA, POLγB, TFAM, TFB1, and TFB2 [197, 216]. As both the ICM cells and ESCs actively express pluripotent factors, Oct4, Sox2, and Nanog, their high levels correlate with low levels of mtDNA replication machinery [208, 217]. Main activation of mtDNA replication and transcription occurs at the later stages of embryonic development and during differentiation of ESCs.
Interestingly, the retinoic acid (RA)-induced mESCs display faster differentiation onset, higher expression of POLγ A, POLγB, and TFAM, and earlier increase in mtDNA copy number but more rapid downregulation of pluripotent genes Oct4 and Nanog, compared to spontaneously differentiated mESCs [218, 219]. Consistently, the RA-induced differentiation of ESCs has led to upregulation of mtDNA genes encoding OXPHOS subunits [220, 221]. RA appears to mediate its effect through activation of PGC-1α, a master regulator of mitochondrial biogenesis and function [222]. Taken together, these findings also suggest that loss of pluripotency, cell-fate commitment, and switch to aerobic mitochondrial respiration represent sequential events during differentiation of ESCs.
Mitochondrial Metabolism
The early embryos are adapted to hypoxic environment and consume relatively little oxygen and predominantly glucose, pyruvate, lactate, and amino acids [223, 224]. Although glycolysis is a less efficient ATP-generation process than OXPHOS, in the early embryos approximately 90% of glucose is metabolized via glycolysis [225]. The critical role of the glycolytic mode in preimplantation embryos has been confirmed by RNA interference attenuation of glucose transporter that resulted in increased apoptosis in the subsequent stages of embryonic development [226].
Undifferentiated ESCs and iPSCs typically exhibit similar prevalence of anaerobic glycolysis as the major energy source [227–231]. As a result these cells require more hypoxic condition and contain significantly lower cellular levels of ATP. It has been reported that lower O2 tensions (3–5% O2) better support the proliferation and maintenance of hESCs in culture than the traditional culture conditions (21% O2) [232]. Consistently, ESCs, including hESCs, prior to the initiation of their differentiation into cardiomyocytes, exhibit the elevated expression of glycolytic enzymes and high glycolytic flux, whereas the expression of ETC subunits and citric acid cycle enzymes is downregulated [197, 227, 233]. Importantly, activation of glycolytic enzyme expression appears to occur prior to the upregulation of pluripotent markers during reprogramming of somatic cells into iPSCs [234, 235]. Accordingly, inhibition of glycolysis results in the reduced reprogramming capacity.
The mammalian target of rapamycin (mTOR) signaling pathway can modulate the speed and efficiency of reprogramming of somatic cells into iPSCs by regulating mitochondrial metabolism and dynamics [231]. Pharmacological inhibition of mTOR leads to a switch from mitochondrial respiration to cytosolic glycolysis and to decrease in expression of PGC-1α, a central regulator of mitochondrial biogenesis [236, 237]. mTOR inhibition may also stimulate mitochondrial fission during reprogramming of somatic cells into iPSCs inducing mitophagy and resulting in decrease in the number and size of mitochondria [238].
Anaerobic glycolysis supplies sufficient energy for the early embryos and undifferentiated ESCs; however, their differentiation is an energy-demanding process requiring a switch from glycolysis to mitochondrial OXPHOS. The expression of glycolytic enzymes is downregulated, while the expression of OXPHOS subunits and citric acid cycle enzymes is upregulated upon mESC differentiation into cardiomyocytes [227]. Hence, O2 consumption, OXPHOS, and ATP generation are significantly increased following the blastocyst stage, and in differentiating ESCs, mtDNA replication and mitochondrial biogenesis are resumed and mature functional mitochondrial networks are formed [197, 199, 225, 227].
Mitochondrial membrane potential (Δψm) is an essential driving force for mitochondrial ATP synthesis; however, current data on its dynamics during differentiation of ESCs are controversial. Chung et al. [227] have reported that high Δψm of undifferentiated mESCs is significantly decreased during their differentiation into cardiomyocytes, while Saretzki et al. [239] have found no changes in Δψm during differentiation of hESCs. Although this controversy may result from the fact that ESCs from mouse and human have been studied, future careful analysis of Δψm in undifferentiated and differentiating ESCs as well as in iPSCs is needed.
Oxidative Stress in Stem Cells
While anaerobic glycolysis is less energetically efficient compared to mitochondrial respiration, it is not associated with the harmful generation of ROS [240]. Switch in energy metabolism from glycolysis to OXPHOS during differentiation of ESCs can lead to an increase in intracellular levels of a by-product of mitochondrial respiration, ROS. Indeed, low levels of ROS, found in undifferentiated ESCs, are increased upon their differentiation [241]. Accordingly, levels of oxidized proteins, lipids, and DNA have been very low in both undifferentiated hESCs and iPSCs, and they have significantly increased during differentiation into fibroblast-like cells [229].
The antioxidant enzymes, such as Cu/Zn superoxide dismutase (Cu/Zn SOD, also known as SOD1), glutathione peroxidase 1 (GPX1), and peroxiredoxin 1 and 2, are also significantly upregulated upon differentiation of hESCs [195, 241]. However, the upregulation of the antioxidant system apparently does not compensate for the increased generation of ROS due to the activation of aerobic mitochondrial metabolism in differentiating ESCs. Intriguingly, overexpression of mitochondrial antioxidant enzyme SOD2 has been reported to facilitate the differentiation of myoblasts derived from young mice but not from aged mice [242]. Therefore, future investigations have to elucidate whether the antioxidant enzymes or other mitochondrial pathways affect similarly adult and embryonic stem cells.
In addition to ROS-mediated damaging effects, ROS play an important role as a critical signaling intermediate implicated in control of cell proliferation and differentiation [241, 243, 244]. Exposure of mESCs to low levels of H2O2 has induced expression of the cardiac-specific genes, encoding the transcription factors GATA-4, Nkx-2.5, MEF2C, and DTEF-1 and the growth factor BMP-10, and stimulated their cardiovascular differentiation. In contrast, high levels of H2O2 have inhibited the differentiation [241, 245]. ROS-induced cardiomyogenesis of mESCs has been abolished by inhibiting NADPH oxidase, while an upregulation of the NADPH oxidase isoforms NOX2 and NOX4 has enhanced cardiac differentiation of mESCs [245, 246]. Moreover, it has been reported that ROS-mediated signaling appears to play a role at earlier stages of cardiomyogenesis of mESCs, while nitric oxide may be involved at late stages [246].
Mitochondria-Mediated Apoptosis of Stem Cells
One of the important direct effects of oxidative stress and endogenous ROS on stem and progenitor cells is cell death. Accumulation of oxidative damages in mtDNA in differentiating ESCs can lead to mutations in mitochondrial genomes affecting ATP production, cell proliferation, and differentiation resulting subsequently in apoptosis [8, 247, 248]. Excessive ROS generation can impair not only mitochondrial respiration but also Ca2+ homeostasis contributing to initiation of cell death.
In mESCs, ROS induces silent mating type information regulation 2 homolog 1 (SIRT1) to deacetylate acetyllysine residues of the tumor suppressor p53, inhibiting thereby p53 translocation to the nucleus [249, 250]. Accumulation of p53 in mitochondria promotes the release of apoptotic factors, such as APF1, cytochrome c, and Smac/DIABLO, leading to mitochondria-mediated apoptosis of oxidatively damaged cells [251–253]. Furthermore, in hESCs, p53 inhibits expression of one of the central pluripotent factors Nanog [153, 154, 254]. Thus, ROS-induced SIRT1 activation contributes to ESC maintenance by both inducing mitochondria-mediated apoptosis of damaged cells and by attenuating p53-mediated inhibition of Nanog. In addition to p53, two other factors, implicated in mitochondrial apoptotic pathway, Bcl-2 and caspase 3, play the role of mediators in stem cell differentiation [255–257].
Conclusions
Stem cell biology is one of the most rapidly progressing fields in biomedical research. Over the past 30 years, considerable advances in experimental heart stem cell therapy based on animal laboratory research have led to several clinical trials. Various cell types and delivery strategies have been examined in experimental and clinical settings; however, the ideal cell type or cell delivery method for cardiac cell therapy has yet to emerge. Although adult stem cells have currently been used in ∼75% of therapeutic procedures, while hESCs, umbilical, and fetal stem cells have been used in 13, 10, and 2% of treatments, respectively, the debate on allogenic versus autologous is still ongoing [258]. Given the time needed to prepare autologous cells, allogenic cells are the only “off-the-shelf” product, which could be use in acute setting. On the other hand, autologous cells from aging patients may have decreased self-renewal capacity. Moreover, it is conceivable that different cell types as well as different techniques of their delivery may be preferable for therapeutic treatment of different cardiovascular disorders.
Two main properties of stem cells, indefinite self-renewal and pluripotency, determine their use in regenerative medicine. Great recent progress in our understanding the molecular mechanisms of cell cycle control and cardiac development has been made during the past two decades. The intricate regulatory circuitry governing the balance between cell cycle progression and lineage commitment has emerged. Finely tuned interplay of c-Myc, as a licensing factor, with the pluripotency trio Oct4/Sox2/Nanog in cooperation with Klf4, orchestrates expression of myriads of genes. Chromatin-remodeling machinery and regulatory RNAs are also involved in this intricate network.
Capability of self-renewal and differentiation is a prominent feature of so-called cancer stem cells [259]. Cancer stem cells are believed to be the source of various human tumors. Therefore, further insights into the mechanisms underlying self-renewal, pluripotency, and differentiation are essential for the development not only of cell-based therapy but also for cancer therapy.
In the constantly contracting heart, mitochondria perform multiple vital functions ranging from ATP production to regulation of cellular homeostasis and survival. However, our knowledge of a role of mitochondria in the maintenance of stem and progenitor cells as well as during their differentiation into cardiomyocytes is still in its infancy.
Undifferentiated ESCs and iPSCs are characterized by several morphological and metabolic features distinct from differentiated somatic cells. They contain the decreased number of immature mitochondria displaying perinuclear localization. The functional significance of these structural characteristics remains yet to be determined. Levels of mtDNA and factors, implicated in mtDNA maintenance, are also significantly diminished. Expression of glycolytic enzymes is upregulated, whereas expression of subunits of ETC is downregulated resulting in lower O2 consumption and lower levels of intracellular ATP. Thus, undifferentiated ESCs and iPSCs are characterized by anaerobic energy metabolism well adapted to hypoxic conditions, to which early embryos are exposed. Based on these characteristics, a “metabolic state hypothesis,” linking the mitochondrial and cellular energy metabolism to the “stemness” and state of differentiation, has been proposed (Fig. 9.3) [228].
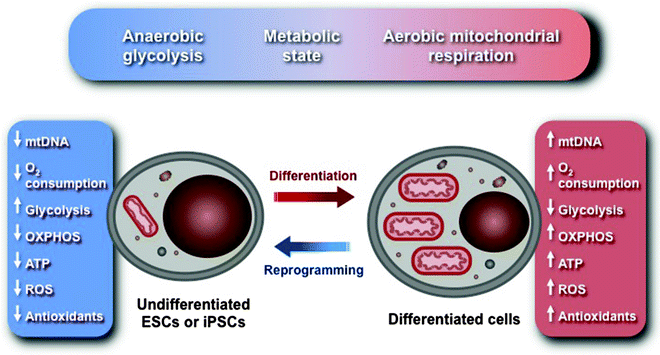
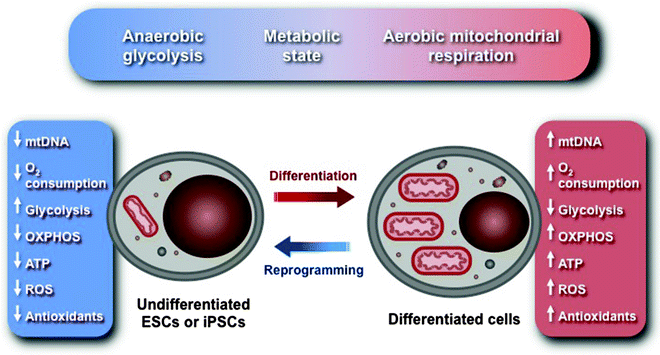
Fig. 9.3
Schematic representation of the “metabolic state hypothesis.” Undifferentiated embryonic stem cells (ESCs) and induced pluripotent stem cells (iPSCs) are characterized by anaerobic energy metabolism well adapted to hypoxic conditions, to which early embryos are exposed. They contain the decreased number of immature mitochondria with poorly developed cristae displaying perinuclear localization. Levels of mitochondrial DNA (mtDNA) and factors, implicated in mtDNA maintenance, are also significantly diminished. Expression of glycolytic enzymes is upregulated, whereas expression of subunits of electron transport chain (ETC) is downregulated resulting in lower O2 consumption and lower levels of intracellular ATP and reactive oxygen species (ROS). Upon ESC differentiation into cardiomyocytes, the expression of glycolytic enzymes is downregulated, while the expression of oxidative phosphorylation (OXPHOS) subunits is upregulated. Hence O2 consumption, OXPHOS and ATP synthesis, and ROS generation are significantly increased, mtDNA replication and mitochondrial biogenesis are resumed, and mature functional mitochondrial networks are formed
Although characteristic mitochondrial morphology, localization, and function have been suggested as markers of self-renewal and pluripotency of stem cells, our knowledge about causal relations between mitochondrial structure and arrangements and bioenergetics, biogenesis, and dynamics is limited. Similarly, while Δψm is a well-known electrochemical driving force that mediates various mitochondrial functions, its dynamics during differentiation of ESCs is a subject of open debate. Further comparative analysis of relationship between Δψm and mitochondrial activities in undifferentiated and differentiating ESCs has to address this important issue.
Importantly, low mitochondrial activity is not only a common feature of stem cells, spermatogonia, and early stage mammalian embryos, but it is also a prominent characteristic of cancer cells. Despite the variability among cancer cells, they are characterized, similar to stem cells, by anaerobic glycolysis as preferred energy-producing pathway and mitochondrial dysfunction [230, 260, 261]. Therefore, insight into the molecular mechanisms underlying remodeling of mitochondrial and cellular bioenergetics during iPSCs reprogramming and tumorigenesis will be mutually beneficial for both stem cell and cancer biology.
Damage-induced activation of the p53 tumor suppressor pathways plays a key role in the maintenance of stem cells. p53 can impair mitochondrial function, affecting ATP synthesis and ROS generation, mediate apoptotic removal of damaged cells, and control the expression of the pluripotent factor Nanog. Emerging evidence suggests a functional interplay between p53-mediated pathways, telomere maintenance, and mitochondrial function [262]. Importantly, the p53-telomere circuit mediates aging process and contributes to age-related diseases. However, how mitochondria are integrated into this circuitry of aging and mechanisms linking the p53-telomere axis to mitochondrial dysfunction and stem cell proliferation and differentiation remains to be elucidated.
Over the past decade we have witnessed an explosion in experimental and clinical studies investigating different cell types and delivery techniques, their efficacy, and safety for therapeutic treatment of cardiovascular disorders. Although benefits so far are modest and less impressive than were observed in animal models, the potential of cell-based therapy remains undiminished. Many fundamental and technical questions still remain to be fully addressed before stem cells enter the clinical realm. Further insights gleaned from studying mitochondrial function in stem and progenitor cells will no doubt accelerate the design of realistic clinical cell-based therapy for cardiovascular disorders.
Summary
Stem cells represent undifferentiated cells characterized by the potential for self-renewal and by the unique ability to differentiate into various cell types, so-called pluripotency. Various cell types proposed as candidates for the cell-based repair of damaged heart can be divided into two groups: allogenic and autologous according to their origin. Allogenic cells include embryonic stem cells (ESCs), fetal cardiomyocytes, and umbilical cord-derived cells, whereas autologous or adult stem cells include bone-marrow- and adipose-derived stem cells, skeletal myoblasts, resident cardiac progenitor cells (CPCs), and induced pluripotent stem cells (iPSCs).
ESCs are the prototypical stem cells, which give rise to all cells of three embryonic germ layers. They exhibit indefinite self-renewal capacity and have the highest developmental potential, including the ability to differentiate into cardiomyocytes. Fetal cardiomyocytes were one of the first cells proposed for the therapeutic use in heart repair. Although ESCs and fetal cardiomyocytes, transplanted into mouse ischemic or failing hearts, form stable grafts and improve cardiac function, they have several potential disadvantages: as allogenic cells, they induce immunological rejection and their therapeutic use raises the ethical and legal concerns. Finally, ESCs can form teratomas after transplantation into various tissues, including the heart.
Cord blood contains a significant amount of non-hemopoietic immunologically naïve stem cells. They typically do not express HLA class II antigens, and therefore, their transplantation reduces the risk of immunological rejection. Human cord blood mononuclear cells (hUCBCs), containing hematopoietic and mesenchymal stem cells, are presently used for treatment of patients with acute leukemia.
Compared to allogenic stem cells, autologous adult stem cells are characterized by a lower capacity to self-renew and to differentiate into various cell types. On the other hand, they exhibit the reduced risk of tumor formation and do not face immunological challenges.< div class='tao-gold-member'>Only gold members can continue reading. Log In or Register a > to continue
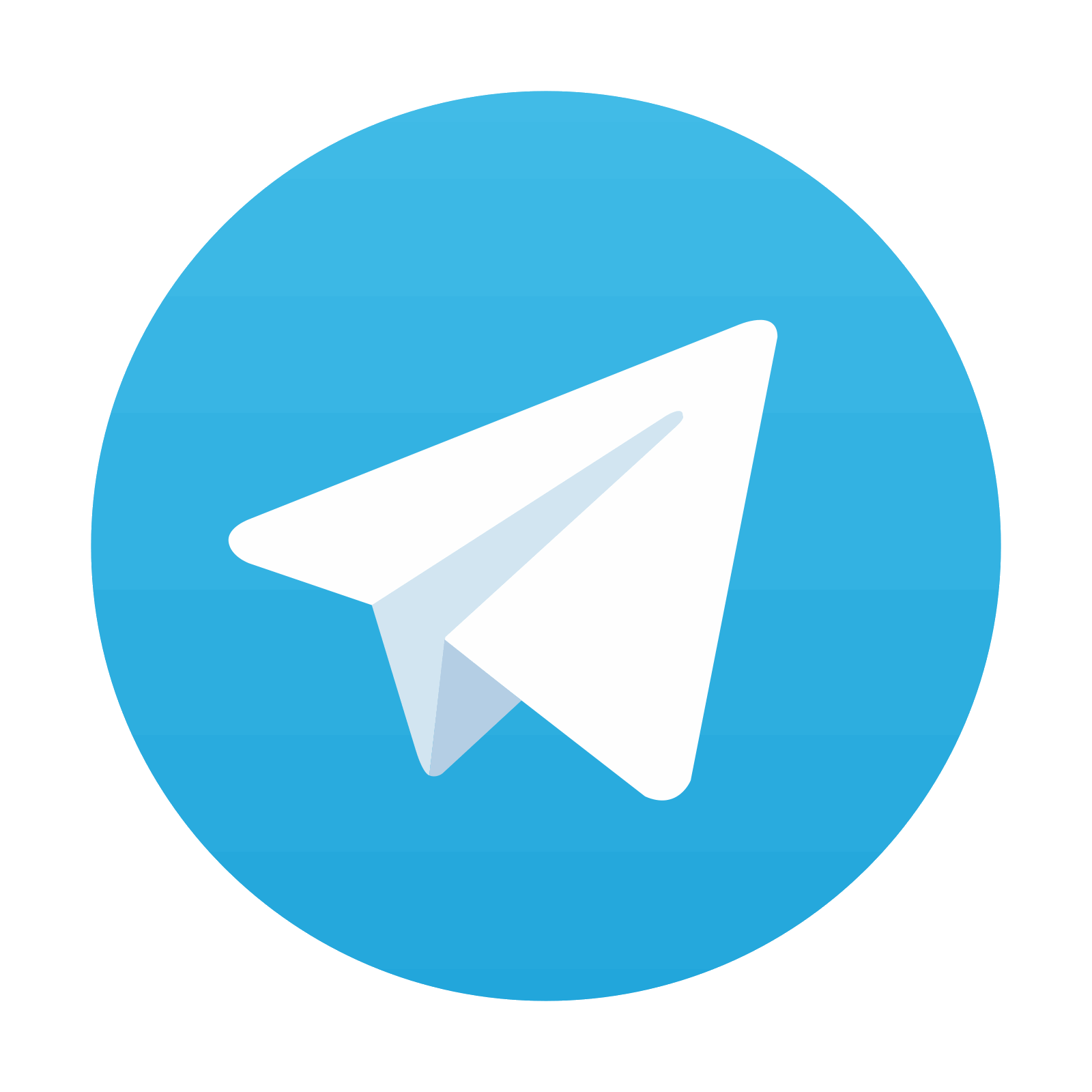
Stay updated, free articles. Join our Telegram channel
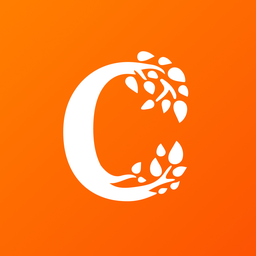
Full access? Get Clinical Tree
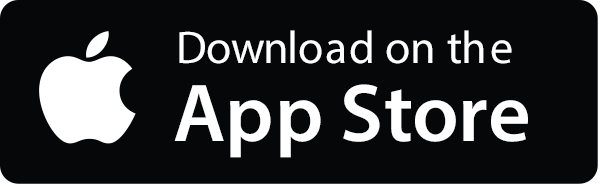
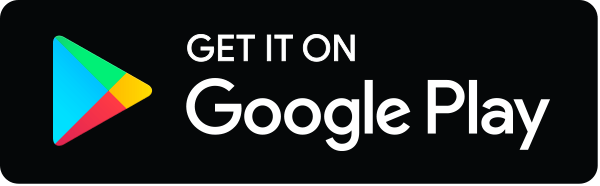