Right Ventricular Anatomy
- Judy R. Mangion, MD
- Scott D. Solomon, MD
Historically, the echocardiographic assessment of diseases affecting the right ventricle (RV) has lagged behind that of the left ventricle, despite knowledge demonstrating that diseases affecting the right side of the heart have been shown to have important clinical consequences. The geometry of the RV is very complex in normal subjects, and even more complex in diseased states, which makes it especially difficult to assess with two-dimensional (2D) techniques (Video 32.1). The RV has a thin wall and has a circumferential arrangement of myofibers in the subepicardium and longitudinal fibers in the endocardium ( Fig. 32.1 ). The RV assumes a flattened, pear-shaped appearance folded over the left ventricle (LV). It consists of three components: (1) an inlet portion consisting of the tricuspid valve, chordae tendineae, and papillary muscles; (2) a trabecular apical myocardium; and (3) an infundibulum or conus, which encompasses the smooth-walled RV outflow tract, beneath the pulmonic valve ( Fig. 32.2 ).


Coronary flow to the right ventricle
It is important to think about RV anatomy in the context of coronary flow to the RV ( Fig. 32.3 ). Coronary flow to the RV is unique in that it occurs during both systole and diastole. The right coronary artery (RCA) provides predominant flow, supplying the lateral wall through acute marginal branches, and it also supplies the posterior wall and posterior interventricular septum through the posterior descending artery. The anterior wall of the RV is supplied by the conus artery branch of the RCA and by branches of the left anterior descending artery.

Echocardiographic assessment of right ventricular anatomy
It is also useful to think about RV anatomy in segmental terms, similar to the LV. The segments of the RV include an anterior RV, inferior RV, lateral RV, and RV outflow tract (RVOT) ( Fig. 32.4 ). A segmental approach to the evaluation of right ventricular systolic function begins with each of the standard 2D transthoracic views. In the parasternal long-axis view ( Fig. 32.5 , A; Video 32.5, A ), the RVOT is visualized. In the parasternal short-axis view (see Fig. 32.5 , B, and Video 32.5, B ), the anterior free wall, lateral free wall, and inferior free wall of the RV are visualized. In the RV inflow tract view (see Fig. 32.5 , C, and Video 32.5, C ), the anterior free wall and inferior free wall of the RV are visualized. In the standard apical four-chamber view (see Fig. 32.5 , D, and Video 32.5, D ), the lateral free wall and right ventricular apex are visualized. In the subcostal four-chamber view (see Fig. 32.5 , E, and Video 32.5, E ), the inferior free wall of the RV or diaphragmatic surface of the RV is visualized. It should be emphasized that the standard apical four-chamber view optimizes visualization of the left ventricle ( Fig. 32.6 , A; Video 32.6, A ). To optimize the visualization of the RV, the transducer needs to be moved more laterally (see Fig. 32.6 , B, and Video 32.6, B ). This prevents dropout of the lateral free wall of the RV and right ventricular apex.







The extent of right ventricular regional wall motion abnormalities has been shown to correlate with the site of coronary occlusion. Gemayel and co-workers studied 25 patients with clinical evidence of right ventricular infarction who underwent echocardiography and coronary angiography. Video 32.7, A to D illustrate significant hypokinesis of the RVOT, anterior free wall, inferior free wall, and lateral free wall of the RV in a 72-year-old man with presenting symptoms of an acute inferior wall myocardial infarction. Figure 32.7 represents the coronary angiogram in the same patient demonstrating a proximal occlusion of the RCA. This case illustrates that the more proximal the right coronary occlusion, the more extensive the RV infarction. Contrast this clinical scenario with that of another patient who also had presenting symptoms of an acute inferior myocardial infarction (Video 32.8, A, B ). In this patient, on the apical four-chamber view the lateral free wall of the RV contracts normally, whereas the inferior free wall of the RV on the subcostal view is akinetic. The site of RCA occlusion in this patient is distal ( Fig. 32.8 ). This case highlights the importance of subcostal 2D echocardiographic views in the evaluation of patients with suspected RV infarction. If the subcostal view is not routinely obtained, RV infarction may be missed when the RCA occlusion is distal.


IV-injected echocardiographic contrast agents that are capable of opacifying the left ventricle and improving definition of the endocardial border are valuable but underused tools in 2D transthoracic echocardiography. Despite their ability to improve the accuracy and reproducibility of echocardiographic structure and function, they are less often used to define the right ventricular endocardial border. IV saline contrast is a less expensive tool that could also be used to facilitate visualization of the RV, although its effects last only seconds, whereas the echocardiographic contrast agents last several minutes. Imaging the RV with echocardiographic contrast agents requires slower injection of the contrast media to prevent attenuation artifact. Imaging with contrast agents also requires optimizing transducer position for visualizing the RV ( Fig. 32.9 and Video 32.9). These agents are administered using the same preprogrammed settings on the ultrasound machine that are used for visualizing the left ventricle.

Reference values for right ventricular structure
The American Society of Echocardiography recently published guidelines for the echocardiographic assessment of the right side of the heart in adults, including reference values for right ventricular structure ( eFig. 32.10 ). It is important to note that current values are based on large populations or pooled values from several studies and they are not based on body surface area or gender. Reference data have not yet been classified into mild, moderate, or severe categories. More recent publications have indicated that both gender and body surface area (BSA) play an important part in determining normal RV reference ranges. It is likely that future published RV guidelines will include upper and lower reference ranges corrected for both BSA and gender.

The Physiologic Basis of Right Ventricular Echocardiography
- Payal Kohli, MD
- Nelson B. Schiller, MD
The right ventricle (RV), which had been deemed the forgotten ventricle, is now recognized as a central player in cardiovascular function. Its physiology, shape, function, and coronary blood flow are complex and distinct and impose impediments to noninvasive imaging. This chapter reviews the physiology of the RV and describes echocardiographic, functional, and structural correlates.
The RV was first described as more than a passive conduit by Sir William Harvey in 1616, who recognized that the “right ventricle may be said to be made for the sake of transmitting blood through the lungs, not for nourishing them.” In the following centuries, studies focused on the left ventricle (LV) overshadowed studies of the RV. It was not until the 1970s when the RV became fully recognized as a key player in cardiovascular disease states, such as heart failure and pulmonary hypertension. In the final decades of the twentieth century, standard two-dimensional transthoracic echocardiographic (2D TTE) imaging of the RV became a mainstay for its evaluation. Recently, advances in imaging modalities such as three-dimensional echocardiography (3DE TTE) have improved detection and characterization of RV pathophysiologic states.
Evaluation of the right ventricle by echocardiography relies on knowledge of its anatomy and physiology and involves characterization of wall thickness, shape, ventricular cavity size, and regional and global contractile function. A complete RV examination includes both qualitative and quantitative parameters, including RV size, right atrial (RA) size, RV systolic function, and pulmonary hemodynamics.
Structure and anatomy of the right ventricle
The location of the right ventricle in the thorax as the most anterior cardiac structure places it retrosternally and in the near field of the ultrasound beam, thus limiting optimal echocardiographic imaging windows and resolution ( eFig. 33.1 , top right ). There are several anatomic features that distinguish the RV from the LV ( Table 33.1 ). These include (1) relative apical displacement of the tricuspid valve (TV) compared with the mitral valve (MV), (2) the presence of bands and coarse apical trabeculations, (3) the presence of more than three papillary muscles, and (4) a trileaflet TV with septal papillary muscle attachments.


Right Ventricle * | Left Ventricle * | |
---|---|---|
Structure | Thin compacta, heavily trabeculated cavity | Thicker compacta |
Shape | Crescentic with triangular base | Truncated ellipse |
End-diastolic volume | 75 ± 13 (49-101) | 66 ± 12 (44-89) |
Mass, g/m 2 | 26 ± 5 (17-34) | 87 ± 12 (64-109) |
Wall thickness, mm | 2-5 | 7-11 |
Ventricular pressures, mm Hg | ||
Systolic | 25 (15-30) | 130 (90-140) |
Diastolic | 4 (1-7) | 8 (5-12) |
RVEF, % | > 40-45 | > 50 |
Ventricular elastance (Emax), mm Hg | 1.30 ± 0.84 | 5.48 ± 1.23 |
Afterload resistance, WU | 0.88 (0.25-1.63) | 13.75 (8.75-20) |
Stroke work index, g/m 2 per beat | 8 ± 2 | 50 ± 20 |
Major vector of contraction | Longitudinal | Circumferential and longitudinal |
* Parentheses indicate range of values. RVEF , Right ventricular ejection fraction.
Anatomically, the RV may be separated into three unique components: (1) the RV inlet, which consists of the tricuspid valve (TV), chordae tendineae, and papillary muscles (PMs); (2) the RV body, which is made up of the highly trabeculated apical myocardium; and (3) the smooth outlet conus (also known as the infundibulum). The positioning of the RV places its body as the most rightward cardiac structure and the end of the outflow tract as the most leftward. Therefore a single 2D sector does not encompass the entire ventricle. The anterior location and thin walls of the RV mandate the use of the transducer with the highest available carrier frequency that permits adequate penetration. Most often, the use of higher frequencies, which are optimal for RV imaging, are less successful in imaging the left ventricle. Therefore the RV tends to be imaged at suboptimal resolution (see eFig. 33.1 , top right ).
The RV inlet can be best imaged on the RV inflow (RVI) view (see eFig. 33.1 , left, third from top ), which allows for visualization of the tricuspid annular plane and can be useful in identifying congenital lesions involving the annulus and the TV; these include prolapse, vegetations, and Ebstein anomaly. In most 2D TTE tomographic planes, only two out of three of the tricuspid valve leaflets are visualized, and multiple views are needed to adequately image all chambers. In the RVI view, the anterior and posterior leaflets are best visualized, whereas the apical four-chamber (A4C) view (see eFig. 33.1 , right, second from top ) allows observation and characterization of the anterior and septal leaflets.
The RV body can also be fully imaged on 2D TTE. From a segmental point of view, it is also useful to divide the chamber into its respective anatomic walls (anterior, lateral, inferior, basal mid, and apical). This anatomic classification allows for localization of RV pathologic states, such as occlusion of the right coronary artery, which can result in localized right ventricular infarction ( Fig. 33.2 ). The thin but variable thickness of the walls is an additional factor in segmental susceptibility to ischemia and infarction. In the standard A4C view of the body of the RV, the basal wall, lateral wall (also known as the “free wall”), and apical segments are readily visualized, whereas the RVI view (see eFig. 33.1 , left, third from top ) allows for visualization of the inferior wall of the RV and the anterior and posterior leaflets of the TV. The parasternal short-axis (PSAX) view at the base of the heart allows visualization of the right ventricular outflow tract (RVOT), along with the anterior and lateral cusps of the pulmonic valve (PV) (see eFig. 33.1 , left panel, third from bottom ).

Similar to the division of its walls, the trabeculations of the ventricle are subdivided into three anatomically distinct bands: parietal, septomarginal, and moderator. The crista supraventricularis (CSV) consists of the parietal band and the infundibular septum, and the septomarginal band is continuous with the moderator band ( Fig. 33.3 ). The CSV is an important anatomic marker of RV dimensions that also serves multiple other functions, including narrowing of the TV annulus during systole. In rare cases, the conus portion of the septomarginal band may hypertrophy, cause dynamic systolic obstruction, and create a double-chambered RV. This condition is recognized on echocardiography by a high-velocity systolic jet arising in the mid–RVOT, a normal pulmonary valve, elevated RV systolic pressure by tricuspid regurgitation (TR) jet, and right ventricular hypertrophy. Double-chambered RV is caused by dynamic muscular obstruction in the RVOT resulting in a dynamic gradient. This condition is sometimes encountered in ventricular septal defects that have spontaneously closed and may have protected the pulmonary circuit from pulmonary hypertension. The moderator band also contains the ramus limbi dextri branch of the RCA (see later).

Unlike the LV, where the MV and aortic valve (AV) are in fibrous continuity, the TV and PV are anatomically separated by the ventriculoinfundibular fold, which creates a spatial boundary that may have physiologic significance. For example, endovascular infections can spread directly from the mitral to aortic valves but this is much less common on the right side because of the presence of ventriculoinfundibular fold. The moderator band, when particularly complex, may also connote RV dysplasia.
The geometry of the right ventricle is also complex. Unlike the ellipsoid LV, the RV is triangular when viewed from the side and crescentic when viewed in cross section (see eFig. 33.1 , top right, and Table 33.1 ). This complex three-dimensional shape complicates the echocardiographic quantitation of RV size and ejection fraction. Despite these impediments, there are options for global quantitation of RV function, and these include RV ejection fraction and fractional area shortening from the four-chamber view of RV body, tricuspid annular plane systolic excursion (TAPSE) ( Fig. 33.4 , A ), and RV dP/dt from the acceleration of the tricuspid regurgitation signal. In addition, Doppler tissue imaging (see Fig. 33.4 , B ) may also describe the systolic velocity of the tricuspid annulus (S′) or TAPSE, another surrogate for right ventricular function.

Whereas the difference between mathematical volume of an ellipsoid in systole and diastole can be used to estimate the LV ejection fraction (EF), the estimation of the RV systolic function is more challenging. Compared with the LV, the base-to-apex shortening contributes more to RV emptying (see Table 33.1 ). The body of RV volume and hence EF can be estimated by measuring the body of the RV (i.e., the RV in the four-chamber view) during systole and diastole and applying either the fractional area change or area-length volume estimation to calculate RVEF. Importantly, only the compact muscle layers of the ventricle should be included in this measurement, and the trabecular layer should be systematically excluded. Because the infundibulum can account for 25% to 30% of RV volume, awareness of its absence should attend the analysis of measurements of the RV body. Owing to the complex geometry of the RV when qualitatively evaluating RV size by 2D TTE, multiple complementary views should be considered before suggesting RV enlargement ( Fig. 33.5 ). Note that the image of the body of the RV in the four-chamber view often includes an outpouching that is a normal anatomic structure known as the acute margin of the heart . The American Society of Echocardiography reference limits for normal RV linear dimensions in the apical four-chamber view are basal RV diameter, 2.0 to 2.8 cm; mid-RV diameter, 2.7 to 3.3 cm; and base-to-apex length, 7.1 to 7.9 cm. Severe enlargement is characterized by a basal diameter of at least 3.9 cm, mid diameter of at least 4.2 cm, and length at least 9.2 cm. Normal RVOT diameter is 2.5 to 2.9 cm above the aortic valve, and severe dilatation is at least 3.6 cm. The American Society of Echocardiography published reference ranges for the apical four-chamber RV end-diastolic and end-systolic areas are 11 to 28 cm 2 and 7.5 to 16 cm 2 , respectively. Echocardiographic measures of RV size are significantly different in men and women, as demonstrated in a study using 2D and 3D echocardiography. In one study, the authors found that RV end-diastolic volume using 3D echocardiography was larger in men than women (129 ± 25 versus 102 ± 33 mL, P < .01).

In addition to RV function and dimensions, the mass of RV also poses a clinical challenge. The RV mass is <SPAN role=presentation tabIndex=0 id=MathJax-Element-1-Frame class=MathJax style="POSITION: relative" data-mathml='1/6′>1/61/6
1 / 6
that of the LV, whereas its volume is larger, and the mass is asymmetrically distributed. RV wall thickness can be measured in diastole, from the subcostal view (using either M-mode or 2D TTE) or in the left parasternal view. Agitated saline or microbubble contrast may be helpful in measuring wall thickness by distinguishing the compact from the trabecular muscle. Standard practice calls for using RV free wall thickness greater than 5 mm to define RV hypertrophy, but quantification of total RV mass has not been satisfactorily performed with 2D TTE. During normal loading conditions, the RV retains its crescentic shape. However, in the setting of pressure or volume overload because of ventricular interdependence, the RV may hypertrophy and become more circular or spheroid, whereas the LV may assume a crescentic shape. Such geometric transformations may alter the mathematical assumptions that are used in normally shaped hearts to extrapolate EF from linear dimensions to become particularly inaccurate once ventricular remodeling has occurred. Two-dimensional biplane measurements from apical views continue to provide accurate information about volume and function in this setting.
Ejection and function of the right ventricle
A discussion of the contraction of the RV and its hemodynamic correlates is informed by considering the myocyte configuration unique to this thin-walled ventricle. There are two layers of muscle fibers in the RV wall (superficial and deep) with a complex overlapping pattern that forms a three-dimensional network. The superficial muscle layer is parallel to the atrioventricular groove and the right coronary artery, whereas the deep fibers are longitudinally aligned from base to apex. The superficial RV fibers are continuous with those of the LV, resulting in continuity between the ventricles. The functional consequences of this continuous layer include coordination of the RV and LV, ventricular interdependence, and traction on the RV free wall caused by LV contraction.
Although the normal RV operates at lower pressure than the LV, the ventricles are connected in series, and their effective stroke volume must be equal. There are many factors that maintain this equality, including the pericardium (so-called fifth chamber), the interatrial and interventricular septa, and great veins and pulmonary veins (Dr. John Tyberg, personal communication). For the echocardiographer, the motion and position of the interatrial septa during respiration is one among many examples of how small pressure and volume changes in the atria during the respiratory cycle are constantly modulating interventricular output.
The contraction of the RV occurs in a sequential fashion, beginning with the trabeculations and ending with the contraction of the conus, about 25 to 50 milliseconds apart. During RV systole, the free wall moves inward, then the long axis shortens, and the base descends towards the apex. Because of the deeper longitudinal fibers, the RV shortens longitudinally more than it shortens horizontally, which is different from the LV. A higher surface area–to-volume ratio of the RV allows for less inward motion than the LV for same volume ejected.
The fiber orientation of the RV musculature makes the longitudinal vector of its contraction the most important; this is appreciated in real-time imaging by the highly visible descent of the RV base (also known as the movement of the tricuspid annulus toward the apex ) that occurs during systole. This motion is best appreciated in the apical and subcostal views of the RV. Basal descent may be measured as a surrogate for RV systolic function and is also known as TAPSE. TAPSE is quantitated as a linear M-mode measurement through the lateral annulus of the tricuspid valve; TAPSE greater than 1.6 is consistent with normal RV systolic function. A failing RV, such as in end-stage cor pulmonale or severe pulmonary hypertension, rarely has a TAPSE that measures above 1 cm. Although TAPSE measures only longitudinal function and is a focal measurement, it has shown good correlation with techniques estimating RV global systolic function, such as radionuclide-derived right ventricular ejection fraction (RVEF).
Right ventricular hemodynamics
As with the LV, RV function is based on preload, contractility, and afterload, and each of these will be sequentially discussed in the following sections.
RV Preload
The RV has a thin wall and operates at low filling pressure, making it very sensitive to changes in preload. Physiologically, this sensitivity becomes apparent during exaggerated respiration or when pericardial restraint is increased. For this reason, the free wall of the RV, in the absence of pulmonary hypertension, collapses during states of pericardial tamponade. This collapse is in proportion to the elevation in intrapericardial pressure and is respirophasic, reflecting increased sensitivity to the waxing and waning of caval filling. This is in turn dictated by the respiratory cycling of the thoracic pump as it overcomes or succumbs to inflow obstruction imposed by elevated intrapericardial pressures. The left ventricle has a thick wall and higher filling pressure, so it resists collapse from elevated intrapericardial pressures during early tamponade. As tamponade worsens, however, transmural pressure rises and the left atrium may also phasically collapse.
RV Contractility
A first step in evaluating right ventricular contractile function is visual inspection of the real-time two-dimensional echocardiogram. Because the wall of the RV is thin, careful adjustments of instrument gain and settings and judicious selection of transducers may be needed to accurately detect RV inward systolic motion or wall thickening. In addition, the RV is extremely sensitive to loading conditions. For example, a high pulmonary vascular resistance (afterload) may affect the EF and contractility of the RV much more than it would impact the LV. Given that the RV and LV stroke volumes have to be identical in the absence of a shunt, decrease in RV stroke volume may significantly decrease the preload of the LV ( Table 33.2 ), thus diminishing its volume and obscuring preexisting pathology as well as diminishing cardiac output. Therefore it is important to note comprehensive RV hemodynamics in any complete echocardiographic assessment.
Controls | Cor Pulmonale | |
---|---|---|
Right ventricle/left ventricle | 0.6 ± 7 | 1.1 ± 0.6 |
Right atrium/left atrium | 0.8 ± 0.3 | 1.3 ± 0.7 |
Pressure-volume loops are particularly helpful for understanding the complex interplay of RV hemodynamics as they contribute to RV function. The slope of the end-systolic pressure-volume relationship is defined as the elastance. Elastance is a measure that is relatively independent of load and therefore a reliable index of contractility. The end-systolic volume index of the LV, an expression of elastance, is a relatively load-independent indicator of LV function and offers independent prognostic information about adverse cardiovascular outcomes such as mortality and heart failure in patients with coronary artery disease. However, because of geometric contraints, RV end-systolic volume is difficult to measure accurately by 2D TTE, and a noninvasive expression of its elastance is not readily available. Hopefully, future research in this area, particularly with 3D volumes of the RV, will provide this potentially valuable clinical information ( Fig. 33.6 ).

Right ventricular stroke work index (SWI), a combined expression of the pressure and volume work done by the right ventricle, is another useful parameter. It can be calculated by subtracting right atrial pressure from mean pulmonary artery pressure and multiplying this difference by stroke volume index. Because of the difference in structure and contractile properties of the LV and RV, their relative stroke work indices are quite different, whereby the RV SWI is only approximately 15% of LV SWI.
The systolic and diastolic right ventricular “volumes” (minus the RVOT volume) is computed by the area length algorithm, using values obtained by tracing the outline of the cavity in the four-chamber view in systole and diastole. The RVEF is calculated as follows: RVEF = (RV end-diastolic volume − RV end-systolic volume) ÷ RV end-diastolic volume. The area length method correlates reasonably well with the EF obtained by radionuclide blood pool imaging. One study was able to replicate quantitative angiography of the RV volume in children by adding the area length volume of the apical view to the volume from the subcostal view. An alternative method of measuring RV contractile function is the RV fractional area change as measured in the apical four-chamber view. Normal RV fractional area change is 32% to 60%, mildly reduced is 25% to 31%, moderately reduced is 18% to 24%, and severely reduced is 17% or less. With emerging technologies, 3D TTE has been validated using the disk summation method as a way to calculate RVEF, but the methodology is still being refined.
RV Afterload
The RV has heightened sensitivity to increased afterload for several reasons: (1) coronary flow is more vulnerable, and increases in pressure can readily lead to RV ischemia (see RV perfusion, later); (2) the RV has a thin wall, so wall stress, which is estimated by Laplace law (and is inversely proportional to twice wall thickness), increases more rapidly with pressure increase than in the LV. Normally the resting peak systolic pulmonary pressure achieved by the RV is less than 30 mm Hg, but this value varies by age and cardiac output. With exercise, the pulmonary pressure may rise as high as 40 mm Hg in unconditioned normal individuals and as high as 55 mm Hg in athletes or persons over age 65 years. Characteristically, normal systolic pulmonary pressure rises slowly through grades of cardiac output that attend increasing exercise levels. Rapid increases in pressure are more characteristic of a pathologic response. Thus the healthy right ventricle has considerable reserve as long as the pressure load increases slowly and is not accompanied by elevated pulmonary vascular resistance. Abrupt increases in pulmonary pressures are poorly tolerated by the thin-walled RV because the wall stress increases rapidly. Examples of situations in which this intolerance is manifest are acute pulmonary embolism and the abrupt dilation of a transplanted heart when the recipient has underlying elevated pulmonary vascular resistance. Deterioration of RV function in these circumstances is accompanied by rapid dilation of the chamber and by a sudden drop in contractile function. One feature of pulmonary embolism that affords insight into the vulnerability of normal RV function is the segmental loss of RV midwall function that is said to be a diagnostic feature of major pulmonary embolism. We theorize that an abrupt rise in pulmonary pressure and subsequent oxygen demand of the RV myocardium is likely to cause midwall ischemia because the timing of right coronary flow is, contrary to left coronary flow, systolic-dominant. An acute elevation of RV wall stress may markedly impair right coronary blood flow, especially at the midwall. The rise in troponin and the location of the wall motion in acute pulmonary embolism appear to support this pathophysiologic explanation.
The use of Doppler to determine pulmonary artery pressure is a mainstay of current echocardiography practice. The first step is the demonstration of tricuspid regurgitation by color flow Doppler in the A4C view ( Fig. 33.7 ). Then, the continuous wave beam is placed across the jet, and the peak velocity is used to calculate the peak gradient between the right atrium and right ventricle with the Bernoulli equation: Peak gradient (mm Hg) = 4 × Peak velocity. Provided that there is no pulmonary stenosis, this gradient added to the RA pressure is equal to peak systolic pulmonary artery pressure. RA pressure is determined by the respiratory behavior of the inferior vena cava (IVC). An alternative method of estimating RA pressure has been published by the American Society of Echocardiography and is as follows. For an IVC diameter of 2.1 cm or less that collapses more than 50% with a sniff, a normal RA pressure of 3 mm Hg is assigned. For an IVC diameter of at least 2.1 cm that collapses less than 50% with a sniff, an elevated RA pressure of 15 mm Hg is assigned. In indeterminate cases in which the IVC diameter and collapse do not fit this paradigm, an intermediate value of 8 mm Hg is assigned. Additional information to validate RA pressure may be obtained from Doppler imaging of the hepatic vein. Normal RA pressure (less than 5 mm Hg) is assumed if the hepatic vein is systolic-dominant, and low RA pressure (2 mm Hg or negative) is assumed if flow is continuous. If the IVC is not visualized, skilled sonographers may image the superior vena cava (SVC) and obtain the flow profile of pulsed wave Doppler (PWD) seeking the same flow patterns seen in the hepatic vein. Another method of judging RA pressure is to observe the curvature and respiratory responses of the interatrial septum. The chamber with the higher pressure will dictate the curvature. Usually, when the septum is bidirectional, the pressure in both chambers is low.
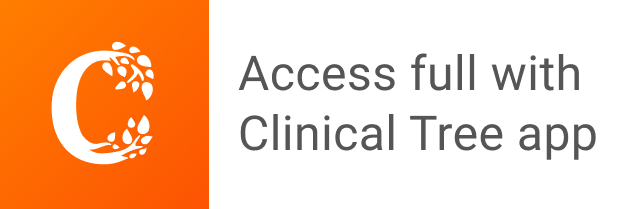