General Principles of Echocardiography
- Frederick W. Kremkau, PhD
Echocardiography is sonography of the heart. Sonography comes from the Latin sonus (sound) and the Greek graphein (to write). Diagnostic sonography is medical real-time, two-dimensional (2D) and three-dimensional (3D) anatomic and flow imaging using ultrasound. Ultrasound is sound of frequency higher than what humans can hear. Frequencies used in echocardiography range from about 2 MHz for adult transthoracic studies to about 7 MHz for higher-frequency applications such as harmonic imaging and pediatric and transesophageal studies. Ultrasound provides a noninvasive view of the heart. Echocardiography is accomplished with a pulse-echo technique. Pulses of ultrasound, two to three cycles long, are generated by a transducer ( Fig. 1.1 ) and directed into the patient, where they produce echoes at organ boundaries and within tissues. These echoes then return to the transducer, where they are detected and presented on the display of a sonographic instrument ( Fig. 1.2 ). The ultrasound instrument processes the echoes and presents them as visible dots, which form the anatomic image on the display. The brightness of each dot corresponds to the echo strength, producing what is known as a grayscale image . The location of each dot corresponds to the anatomic location of the echo-generating object. Positional information is determined by knowing the path of the pulse as it travels and measuring the time it takes for each echo to return to the transducer. From a starting point at the top of the display, the proper location for presenting each echo is determined. Because the speed of the sound wave is known, the echo arrival time can be used to determine the depth of the object that produced the echo.


When a pulse of ultrasound is sent into tissue, a series of dots (one scan line, data line, or echo line) is displayed. Not all of the ultrasound pulse is reflected from any single interface. Rather, most of the original pulse continues into the tissue and is reflected from deeper interfaces. The echoes from one pulse appear as one scan line. Subsequent pulses go out in slightly different directions from the same origin. The result is a sector scan (sector image), which is shaped like a slice of pie ( Fig. 1.3 ). The resulting cross-sectional image is composed of many (typically 96 to 256) of these scan lines. For decades, sonography was limited to 2D cross-sectional scans (or slices) through anatomy such as that in Figure 1.3 . 2D imaging has been extended into 3D scanning and imaging, also called volume imaging, as described in Chapter 2 . This requires scanning the ultrasound through many adjacent 2D tissue cross sections to build up a 3D volume of echo information, like a loaf of sliced bread ( Fig. 1.4 ). In addition to anatomic grayscale imaging, stationary beam, M-mode presentations provide depth versus time recordings of moving objects ( Fig. 1.5 ).



Transducer
The transducer used in echocardiography is a phased array that electronically steers the ultrasound beam in the sector format. It is energized by an electrical voltage from the instrument that produces the outgoing ultrasound pulse. The returning echo stream is received by the transducer and converted to an echo voltage stream that is sent to the instrument, ultimately appearing on the display as a scan line. This process occurs a few thousand times per second (called the pulse repetition frequency [PRF]). A coupling gel is used between the transducer and the skin to eliminate the air that would block the passage of ultrasound across that boundary. Transducers are designed for transthoracic and for transesophageal imaging (see Fig. 1.1 ). The latter provides a shorter acoustic path (with less attenuation, allowing higher frequency and improved resolution) to the heart that avoids intervening lung and ribs.
Instrument
An echocardiographic instrument has a functional block diagram as shown in Figure 1.6 . The beam former drives the transducer and receives the returning echo streams, amplifying (this is called gain ) and digitizing them. Attenuation compensation occurs in the reception side of the beam former. The signal processor, among other functions, detects the strength (amplitude) of each echo voltage. Echo amplitudes are stored as numbers in the image memory, which is part of the image processor. Upon completion of a single scan (one frame of a real-time presentation), the stored image is sent to the display. The display is a flat-panel screen, now common in computer monitors and television sets. The echo information is sent into the image memory in ultrasound scan lines in sector format, but it is read out and sent to the display in horizontal display line format, with each horizontal line on the display corresponding to a row of echo data in the image memory.

Artifacts
In imaging, an artifact is anything that does not correctly display the structures or functions (tissue motion and blood flow) imaged. An artifact is caused by some problematic aspect of the imaging technique. They can hinder correct interpretation and diagnosis. These artifacts must be prevented or handled properly when encountered.
Some artifacts are produced by improper equipment operation or settings (e.g., incorrect gain and compensation settings). Other artifacts are inherent in the sonographic methods and can occur even with proper equipment and technique. The assumptions inherent in the design of sonographic instruments include the following:
- •
Sound travels in straight lines
- •
Echoes originate only from objects located on the beam axis
- •
The amplitude of returning echoes is related directly to the reflecting or scattering properties of distant objects
- •
The distance to reflecting or scattering objects is proportional to the round-trip travel time at a speed of 1.54 mm/μs

Safety
Information derived from in vitro and in vivo experimental studies has yielded no known risks in the use of echocardiography. Thermal and mechanical mechanisms have been considered but do not appear to be operating significantly at diagnostic intensities. Experimental animal data have helped to define the intensity–exposure time region in which bioeffects can occur. However, differences, physical and biological, between the two situations make it difficult to apply results from one risk assessment to the other. In the absence of known risk, it is still necessary to remember that bioeffects not yet identified could occur. Therefore, a conservative approach to the medical use of ultrasound is recommended.
Epidemiologic studies have revealed no known risk associated with the use of diagnostic ultrasound. Experimental animal studies have shown that with most equipment, bioeffects occur only at intensities higher than those expected at relevant tissue locations during ultrasound imaging and flow measurements. Thus a comparison of instrument output data adjusted for tissue attenuation with experimental bioeffects data does not indicate any risk. We must be open, however, to the possibility that unrecognized, but not zero, risk may exist. Such risk, if it does exist, may have eluded detection up to this point because it is subtle or delayed, or its incidence is close to normal values. As more sensitive end points are studied over longer periods or with larger populations, such risks may be identified. However, future studies might not reveal any detrimental effects, thus strengthening the possibility that medical ultrasound imaging is without detectable risk. In the meantime, with no known risk and with known benefit to the procedure, a conservative approach to imaging should still be used. That is, ultrasound imaging should be used when medically indicated, with minimum exposure to the patient. Exposure is limited by minimizing both instrument output and exposure time during a study.
Following is the April 1, 2012, American Institute of Ultrasound in Medicine (AIUM) Official Statement on Prudent Use and Clinical Safety:
Diagnostic ultrasound has been in use since the late 1950s. Given its known benefits and recognized efficacy for medical diagnosis, including use during human pregnancy, the American Institute of Ultrasound in Medicine herein addresses the clinical safety of such use: No independently confirmed adverse effects caused by exposure from present diagnostic ultrasound instruments have been reported in human patients in the absence of contrast agents. Biological effects (such as localized pulmonary bleeding) have been reported in mammalian systems at diagnostically relevant exposures but the clinical significance of such effects is not yet known. Ultrasound should be used by qualified health professionals to provide medical benefit to the patient. Ultrasound exposures during examinations should be as low as reasonably achievable (ALARA).The AIUM statement provides an excellent basis for formulating a response to patient questions and concerns. Prudence in practice is exercised by minimizing exposure time and output. Display of instrument outputs in the form of thermal and mechanical indexes (TIs and MIs, respectively) facilitates such prudent use.
In decades of use, there have been no reports of injury to patients or to operators from medical ultrasound equipment. We in the ultrasound community want to maintain that level of safety. In the past, application-specific output limits and the user’s knowledge of equipment controls and patient body characteristics were the means of minimizing exposure. Now more information is available. The mechanical and thermal indexes provide users with information that can be applied specifically to formulate ALARA guidelines. Values of these indexes eliminate some of the guesswork and indicate the actual physiologic effects within the patient and what occurs when control settings are changed. These values make it possible for the user to obtain the best image possible while following the ALARA principle, thus maximizing the benefits and minimizing the risks.
Advanced features and techniques (3D echocardiography, Doppler, tissue Doppler imaging, speckle tracking echo, tissue harmonic imaging) are covered in more detail in this book. Expansion of all the topics covered in this chapter can be found elsewhere.
Three-Dimensional Echocardiography
- Luigi P. Badano, MD, PhD
- Denisa Muraru, MD, PhD
The milestone in the evolution of three-dimensional echocardiography (3DE) from two-dimensional echocardiography (2DE) has been the development of fully sampled matrix array transthoracic transducers based on advanced digital processing and improved image formation algorithms. This allowed the operators to obtain transthoracic real-time volumetric imaging with short acquisition time and high spatial and temporal resolution. Further technologic developments (e.g., advances in miniaturization of the electronics and in element interconnection technology) made it possible to insert a full matrix array into the tip of a transesophageal probe and provide transesophageal real-time volumetric imaging. In addition to transducer engineering, improved computer processing power and the availability of dedicated software packages for both online and offline analysis have allowed 3DE to become a practical clinical tool.
Comparison between 2DE and 3DE ultrasound transducers
The backbone of the 3DE technology is the transducer. A conventional 2DE phased array transducer is composed of 128 piezoelectric elements, each electrically isolated and arranged in a single row ( Fig. 2.1 ). Each ultrasound wave front is generated by firing individual elements in a specific sequence with a delay in phase with respect to the transmit initiation time. Each element adds and subtracts pulses to generate a single ultrasound wave with a specific direction that constitutes a radially propagating scan line ( Fig. 2.2 ). Because the piezoelectric elements area arranged in a single row, the ultrasound beam can be steered in two dimensions: vertical (axial) and lateral (azimuthal). Resolution in the z axis (elevation) is fixed by the thickness of the tomographic slice, which in turn is related to the vertical dimension of piezoelectric elements (see Figure 2.1 ).



Currently, 3DE matrix array transducers are composed of about 3000 individually connected and simultaneously active (fully sampled) piezoelectric elements with operating frequencies ranging from 2 to 4 MHz and 5 to 7 MHz for transthoracic and transoesophageal transducers, respectively. To steer the ultrasound beam in 3DE, a 3D array of piezoelectric elements must be present in the probe; therefore, piezoelectric elements are arranged in a rectangular grid (matrix configuration) within the transducer (see Fig. 2.1 , right ). The electronically controlled phasic firing of the elements in that matrix generates a scan line that propagates radially ( y, or axial direction) and can be steered both laterally ( x, or azimuthal direction) and in elevation ( z, or vertical direction) to acquire a volumetric pyramid of data (see Fig. 2.1 , right ). Matrix array probes can also provide real-time multiple simultaneous 2D views, at high frame rate, oriented in predefined or user-selected plane orientations ( Fig. 2.3 ). The main technological breakthrough that allowed manufacturers to develop fully sampled matrix transducers has been the miniaturization of electronics. Individual electrical connections can now be set for every piezoelectric element, which can be independently controlled, both in transmission and in reception.

Beamforming is a technique used to process signals so that directionally or spatially selected signals can be sent or received from sensor arrays. In 2DE, all the electronic components for beamforming (high-voltage transmitters, low-noise receivers, analog-to-digital converter, digital controllers, digital delay lines) are in the system and consume a lot of power (around 100 W and 1500 cm 2 of personal computer [PC] electronics board area). If the same beamforming approach was applied to matrix array transducers used in 3DE, it would require around 4 kW power consumption and a huge PC board area to accommodate all the needed electronics. To reduce both power consumption and the size of the connecting cable, several miniaturized circuit boards are incorporated into the transducer, so that partial beamforming can occur in the probe ( Fig. 2.4 ). This unique circuit design results in an active probe, which allows microbeamforming of the signal with low power consumption (< 1 W) and does not require connecting every piezoelectric element to the ultrasound machine. The 3000 channel circuit boards within the transducer control the fine steering by delaying and summing signals within subsections of the matrix, known as patches (see Fig. 2.4 ). This microbeamforming allows the number of digital channels in the cable connecting the probe to the ultrasound system to be decreased from 3000 to 128 to 256. If 3000 channels were required, the cable would be too heavy for practical use. Therefore the cable used with 3D probes is the same size as that used for a 2D probe. Coarse steering is controlled by the ultrasound system where the analog-to-digital conversion occurs using digital delay lines (see Fig. 2.4 ).

However, the amount of heat produced by the electronics inside the probe is directly proportional to the mechanical index used during imaging; therefore the engineering of active 3DE transducers should include thermal management.
Finally, new and advanced crystal manufacturing processes allows production of single crystal materials with homogeneous solid-state technology and unique piezoelectric properties. These new transducers produce less heat by increasing the efficiency of the transduction process, which improves the conversion of transmitted power into ultrasound energy and of received ultrasound energy into electrical power. Increased transduction efficiency together with a wider bandwidth results in increased ultrasound penetration and resolution, which both improves image quality and reduces artifacts. Thus power consumption is decreased, and Doppler sensitivity is increased.
Further developments in transducer technology have resulted in a reduced transducer footprint, improved side-lobe suppression, increased sensitivity and penetration, and the implementation of harmonic capabilities that can be used for both gray-scale and contrast imaging. The most recent generation of matrix transducers are significantly smaller than the previous ones, and the quality of 2DE and 3DE imaging has improved significantly, allowing a single transducer to acquire both 2DE and 3DE studies, and record the whole left ventricular cavity in a single beat.
3D Echocardiography physics
3DE is an ultrasound technique, and the physical limitation of the constant speed of ultrasound frequencies in human tissues (approximately 1540 m/sec in myocardial tissue and blood) cannot be overcome. The speed of sound in human tissues divided by the distance a single pulse has to travel out and back (determined by the image depth) results in the maximum number of pulses that can be fired each second without producing interferences. Based on the acquired pyramidal angular width and the desired beam spacing in each dimension (spatial resolution), this number is related to the volume per second that can be imaged (temporal resolution). Therefore, similar to 2DE imaging, there is an inverse relationship between volume rate (temporal resolution), acquisition volume size, and the number of scan lines (spatial resolution) that can be achieved in 3DE. Any increase in one of these factors will cause a decrease in the other two.
The relationship between volume rate, number of parallel receive beams, sector width, depth, and line density can be described by the following equation:
Therefore the volume rate can be adjusted to the specific needs by either changing the volume width or depth. The 3D system allows the user to control the lateral resolution by changing the density of the scan lines in the pyramidal sector too. However, a decrease in spatial resolution also affects the contrast of the image. Volume rate can also be increased by increasing the number of parallel receive beams, but in this way the signal-to-noise ratio and the image quality will be affected.
To put all this in perspective, let us assume that we want to image depth up to 16 cm and acquire a pyramidal volume of 60 × 60 degrees. Because the speed of sound is approximately 1540 m/sec, and each pulse has to propagate 16 cm × 2 (to go out and back to the transducer), 1540/0.32 = 4812 pulses may be fired per second without getting interference between the pulses. Assuming that 1-degree beam spacing in both X and Z dimensions is a sufficient spatial resolution, we would need 3600 beams (60 × 60) to spatially resolve the 60 × 60 degrees pyramidal volume. As a result, we will get a temporal resolution (volume rate) of 4812/3600 = 1.3 Hz, which is practically useless in clinical echocardiography.
The previous example shows that the fixed speed of sound in body tissues is a major challenge to the development of 3DE imaging. Manufacturers have developed several techniques such as parallel receive beamforming, multibeat imaging, and real-time zoom acquisition to cope with this challenge; but in practice this is usually achieved by selecting the appropriate acquisition modality for different imaging purposes (see “ Image Acquisition and Display ”).
Parallel receive beamforming or multiline acquisition is a technique where the system transmits one wide beam and receives multiple narrow beams in parallel. In this way the volume rate (temporal resolution) is increased by a factor equal to the number of the received beams. Each beamformer focuses along a slightly different direction that was insonated by the broad transmit pulse. As an example, to obtain a pyramidal volume that is 90 × 90 degrees and 16 cm deep at 25 volumes per second (vps), the system needs to receive 200,000 lines/sec. The emission rate is around 5000 pulses per second, so the system should receive 42 beams in parallel for each emitted pulse. However, increasing the number of parallel beams to increase temporal resolution leads to increases in size, cost, and power consumption of the beamforming electronics, and decreases in the signal-to-noise ratio and contrast resolution. With this technique of processing the received data, multiple scan lines can be sampled in the amount of time a conventional scanner would take for a single line. However, this occurs at the expense of reduced signal strength and resolution, as the received beams are steered increasingly farther away from the center of the transmitted beam ( Fig. 2.5 ).

Another technique to increase the pyramidal volume and maintain the volume rate (or the reverse, e.g., maintain volume rate and increase the pyramidal volume) is multibeat acquisition. With this technique, a number of electrocardiographic (ECG)-gated subvolumes acquired from consecutive cardiac cycles are stitched together to build up the final pyramidal volume ( Fig. 2.6 ). Multibeat acquisition will be effective only if the different subvolumes have constant position and size; therefore any transducer movement, cardiac translation motion due to respiration, and change in cardiac cycle length will create subvolume misalignment and stitching artifacts ( Fig. 2.7 ).

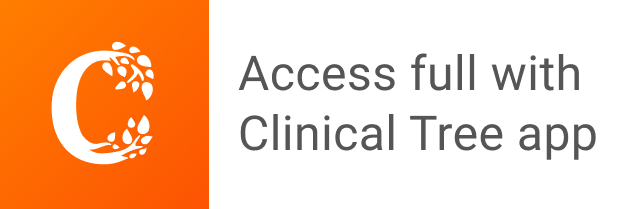